Following the recent measurement of a fundamental parameter of the Standard Model, CERN’s Aleko Khukhunaishvili spoke with The Innovation Platform Editor Maddie Hall about its significance.
In March 2024, at the Moriond Winter Conference, CERN announced that the CMS experiment had achieved the most precise measurement at a hadron collider to date and that this result aligned with the Standard Model (SM).
The effective leptonic electroweak mixing angle, a fundamental parameter of the SM, had been previously measured. However, earlier results were in disagreement, leading to inconclusive support for the SM prediction. This increased precision measurement not only provides stronger support for the SM theory but also unlocks a new level of precision physics at the facility.
To elaborate on the significance of this new parameter, The Innovation Platform Editor, Maddie Hall, spoke with CERN’s Aleko Khukhunaishvili about the CMS experiment and the Standard Model.
Can you briefly summarise the CMS experiment and the purpose of the programme?
The Compact Muon Solenoid (CMS) is a general-purpose, nearly hermetic detector that detects the particles produced in high-energy collisions at one of the high-luminosity interaction points at the LHC. It comprises layers of tracking detectors and calorimeters placed around the interaction point, which are used to identify and reconstruct the energies and directions of nearly all particles produced in the collisions. A standout feature of CMS is its large magnetic field of four tesla, generated by a superconducting solenoid. This substantial magnetic field allows very precise measurements of charged particles and facilitates detailed studies of a large variety of processes occurring during the collisions.
The scientific programme of the CMS experiment is incredibly broad, encompassing the study of the SM processes that involve the newly discovered Higgs-Boson, top quark, and electroweak bosons, among others. With the capacity to collect massive amounts of data, we can execute precision tests to examine theoretical relations between various precise experimental observables predicted in the SM. The new measurement of this electroweak mixing angle falls into this category.
Moreover, CMS is a platform for exploration, used to search for ‘new physics’ event topologies that are not predicted or described by the SM. This includes specific signatures from certain models, like supersymmetry or extra dimensions, that could, for example, provide viable dark matter candidates. Thus, CMS offers a vast potential for discovery and breakthroughs in the understanding of the fundamental laws of nature.
What is the electroweak mixing angle, and how was it measured?
The electroweak mixing angle is one of the key parameters in the SM. It was introduced in the Higgs mechanism, which, in a process known as spontaneous electroweak symmetry breaking, gives mass to the W and Z bosons, the mediators of the weak interaction. This weak mixing angle – or the Weinberg angle relates the masses of the W and Z bosons and also defines the couplings i.e. the strength of the interaction of the Z-boson to quarks and leptons. These couplings are used to define what we call the effective mixing angle, which also depends on the fermion flavour due to radiative corrections.
The experiment used a large number of dilepton events produced in proton-proton collisions to measure the effective leptonic mixing angle. In most of these events, a quark from one of the protons interacts with its anti-quark in the other, producing the Z-boson, which subsequently decays to a lepton pair. If we look at the distribution of the angle of the produced negatively charged lepton relative to the original quark, it has an asymmetry, which is strongly dependent on the value of the leptonic effective mixing angle. We use this measurement of the forward-backward asymmetry to extract the value of this parameter.
Can you explain the Standard Model and its importance?
The Standard Model is the most successful model of particle physics that describes all observed matter and three types of fundamental interactions (electromagnetic, weak, and strong) using only a few building blocks of matter particles and mediators of these interactions. The final formulation was completed in the ‘70s in a combined framework of strong interactions (quantum chromodynamics) and the unified electroweak theory.
Since then, one by one, all of its major predictions have been successfully verified in the experiment – including the discovery of the W and Z bosons at CERN in the early ‘80s and the top quark at Fermilab in 1995. Most recently, the discovery of the Higgs boson at CERN in 2012 was a major triumph, completing all the pieces of the SM.
The obvious question was, what next? When we talk about the SM and all of its successes, there is always an appendix. It is not a complete theory because some questions or phenomena cannot be described, such as the asymmetry between matter and antimatter and the presence of dark matter in the Universe. These phenomena could be explained by a more complete theory or some extension of the SM. Finding these new physics signatures or their indications is now the main goal of the experiments at CERN, and these efforts are actively ongoing.
How is the recent measurement significant for CERN and for particle physics in general?
One reason for its significance is that high-energy hadron colliders and detectors are normally designed for discoveries. They normally probe high-energy regions of phase space not explored before with enormous amounts of hadron collisions. Unlike electron colliders, these produce very crowded events with hundreds or even thousands of background particles emerging from the interaction point: From multiple interactions between the constituents of the protons and from many colliding protons in each event.
This measurement demonstrates that thanks to the powerful CMS detector and particle reconstruction techniques, combined with the dedicated analysis techniques developed for this measurement, we could turn those enormous rates of collisions to our advantage since they also produce a huge number of signal events. These precision measurements are crucial, unlocking a whole new front of indirect searches for new physics beyond the Standard Model at the LHC.
What are the implications of the measurement for our understanding of the Standard Model?
The short answer is that the SM remains successful and resilient.
One motivating factor for this new measurement was that the two most precise previous results obtained in the electron-positron colliders were in disagreement. They were about three standard deviations apart, with each being about two standard deviations up or down from what the Standard Model would predict.
In the subsequent Fermilab Tevatron measurements, the CDF and D0 experiments showed very similar, slightly discrepant results. However, the uncertainties were larger, and so the tension was not significant. The first LHC measurement of this parameter had larger uncertainties but seemed to prefer the lower deviation.
Resolving this discrepancy is very important because if the truth is in the middle, which this most recent measurement prefers, it would mean that the SM prediction is correct. If not, it would indicate new physics. The fact that the new result is in agreement with the SM is significant, and it is also a step towards resolving the conflict presented by the earlier results.
There is definitely still room for improvement and increased precision. As such, we can still only talk about preferences since the uncertainties are not yet small enough to definitively rule one way or another. Planned upgrades to the detector, along with a huge amount of data, will significantly increase the precision of this measurement. Therefore, it is incredibly significant that the experiment has demonstrated the capability to perform this precise measurement, even with the very crowded events from the high-luminosity proton-proton operations.
Could variations in the electroweak mixing angle shed light on the existence of new particles or interactions?
The SM predicts very precise relations between the different experimental observables. As such, the main motivation for this measurement is the potential to shed light on possible new particle interactions. Using various precise experimental inputs, such as the Z-boson mass and width, the Fermi constant from the muon lifetime, the top quark mass, the Higgs-boson mass, etc., the SM can predict the effective leptonic mixing angle very precisely.
Any significant deviation from this prediction would indicate that the SM needs to be altered somehow. Technically, this could be explained by new particles beyond the SM contributing to the radiative corrections of various observables – for example, this parameter or the W boson mass.
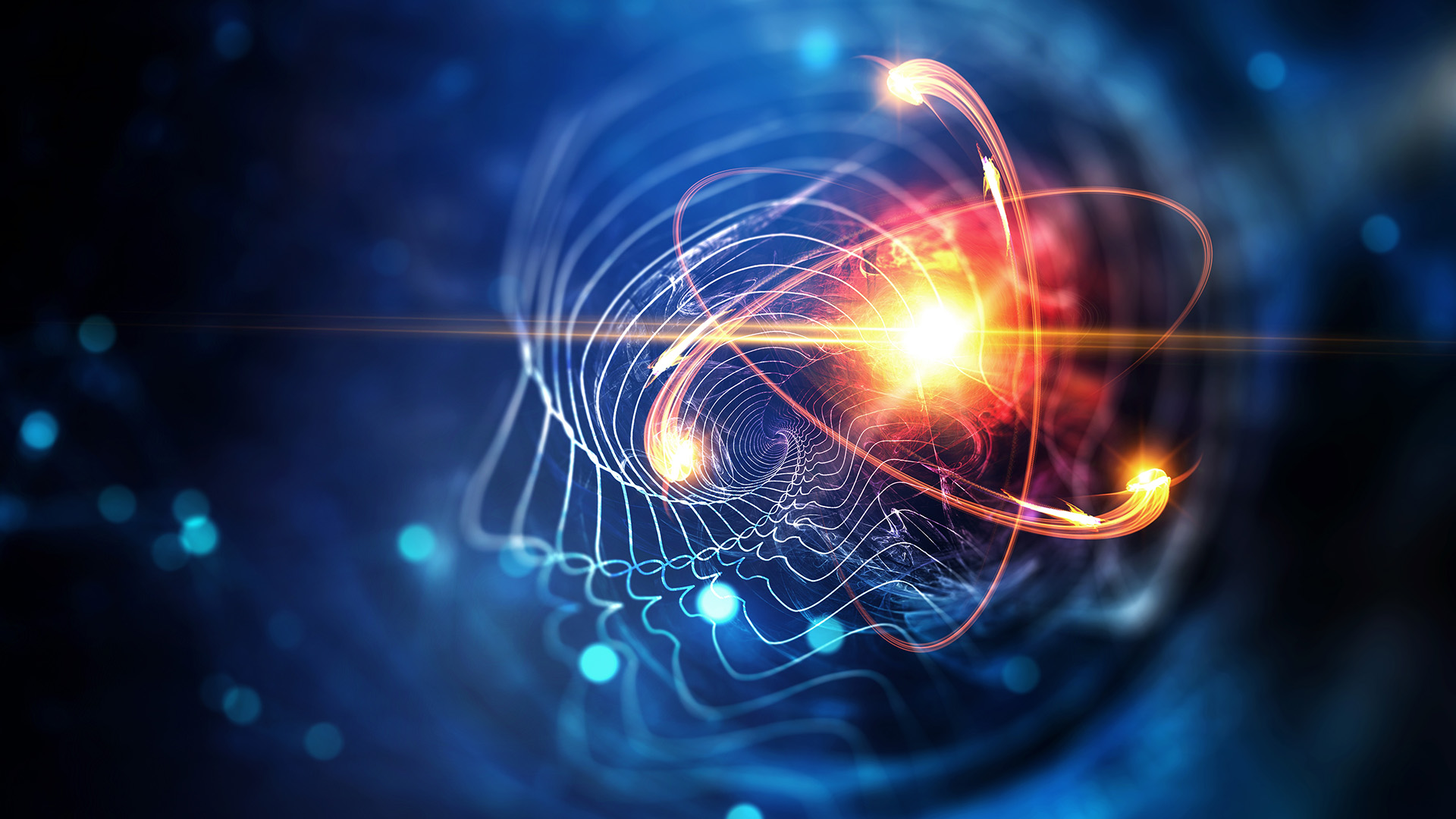
These two variables are closely related, and their measurements, which are the active subjects of the LHC programme, should be interpreted together. Concerning the mass of the W boson, until recently, there was a good agreement between theory and various measurements. In 2022, the CDF very precisely measured the mass of the W boson, a result that shook the community because it disagreed with both the previous measurements and the SM prediction. The relation between the two parameters is such that the value of the W boson mass measured by CDF would prefer a lower value of the leptonic effective weak mixing angle.
What are the challenges in running an experiment such as this, and how did you mitigate or address these?
The main challenge of this measurement in proton-proton collisions is that we have to rely on the knowledge of the distribution of the valance quark contributions to the proton momentum. While this also results in degraded statistical uncertainty, the huge amount of data used overcomes this.
The large uncertainty from the parton distribution functions (PDF) is partially mitigated by measuring the observed forward-backward asymmetry as a function of the dilepton’s mass and rapidity and using this measurement to simultaneously constrain the PDFs in situ.
Additionally, for the first time for CMS, this measurement includes the central-forward dielectron pairs, which are less affected by the PDF uncertainties. This required many dedicated developments for the forward electron reconstruction and identification, which were implemented specifically for this analysis.
A considerable amount of effort went into precise, dedicated measurements of lepton selection efficiencies, lepton momentum scale and resolution calibration, background studies, charge misidentification studies, theory models, and PDF studies. It took more than five years of dedicated effort to work out all the fine details of these various components and estimate the corresponding systematic uncertainties.
How will this parameter benefit and improve future experiments, and how will it be used to reexamine past measurements and experiments?
What we have measured now, this electroweak mixing angle, is already the most precise result achieved at a hadron collider. It is already precise enough to have an important impact on the future global electroweak fits.
In addition, what we measure and publish are the unfolded results of the forward-backward asymmetry. This is important because it will allow anyone to simply reinterpret this measurement without the need to know detector-related effects. For example, an improved value can be obtained from these unfolded results once the new improved PDFs are released. The unfolded measurements will also allow for a simple combination with future weak mixing angle and other electroweak measurements (e.g. the W boson mass) at the LHC.
We also need to make some improvements in preparation for future measurements. This will involve addressing the main limiting factor mentioned earlier, the uncertainties of the PDFs. In this regard, it is important for all LHC experiments to continue providing high-precision differential measurements to be included in the global PDF analyses.
On the experimental side, work will be mainly directed into efficiently collecting huge amounts of dilepton events of various categories during the high-luminosity LHC operation. Far from a trivial task, this will likely require dedicated developments at both the high-level and level-one triggers. There remains some work to be done in order to prepare for the LHC’s ultimate measurement of this parameter.
What’s next for the CMS experiment?
As mentioned, for the high-luminosity LHC operation, the accelerator complex will be upgraded to provide a huge increase in the luminosities of the colliding protons at the interaction points. Accompanied also by significant upgrades of the CMS subdetectors and trigger systems, it will enable a huge increase in the amount of interesting data produced, as much as a factor of 20 compared to now. In addition, for this measurement in particular, extending the pseudorapidity coverage of the tracking detectors will be crucial and significantly improve its precision.
With all these upgrades, physics’ reach in all areas will increase dramatically – precision Higgs Boson and other SM measurements, as well as searches for new physics. It’s safe to say the most exciting times are ahead of us.
Please note, this article will also appear in the 18th edition of our quarterly publication.