Professor Haiyan Gao of Duke University and Dr Zein-Eddine Meziani of Argonne National Laboratory outline the science case for the Solenoidal Large Intensity Device (SoLID) at Jefferson Lab.
Nucleons (protons and neutrons) are the building blocks of atomic nuclei, contributing to more than 99% of the visible matter in the universe. Exploring the structure of matter has been at the forefront of nuclear physics since its birth. Recent advances in such exploration have benefited from innovations and breakthroughs in theory, experiment and computation.
We are now entering a new era of precision studies of quantum chromodynamics (QCD), defined by new instrumentation and facilities measuring novel observables and allowing for precision microscopes to probe the internal structure and dynamics of the nucleon and atomic nuclei with unprecedented precision. The energy-upgraded Continuous Electron Beam Accelerator Facility (CEBAF) at the Thomas Jefferson National Accelerator Facility (Jefferson Lab) and the future Electron-Ion Collider at Brookhaven National Laboratory are two such facilities.
The proposed Solenoidal Large Intensity Device (SoLID)1 at Jefferson Lab will combine the powerful feature of high-intensity CEBAF electron beams with a large acceptance detector enabling three major science goals as highlighted in the Nuclear Science Advisory Committee’s: A New Era of Discovery: The 2023 Long Range Plan for Nuclear Science. These goals include imaging quarks in three-dimensional momentum space inside the nucleon, utilising near-threshold production of charm-anticharm meson systems from the nucleon to extract its mass and scalar profile densities and corresponding radii, and using parity violation in electron scattering to search for new physics beyond the standard model of particle physics.
Three pillars of the SoLID Science:
Proton spin and the confined motion of quarks in a nucleon
Lepton scattering has been a powerful probe, as demonstrated in the 1950s by Hofstadter’s Nobel Prize-winning electron-proton elastic scattering experiment on the electromagnetic structure of the proton. This was followed by another Nobel-winning experiment on inclusive deep inelastic scattering (DIS) by Friedman, Kendall and Taylor, which discovered the underlying quark or partonic structure of the proton. Such measurements provide parton distribution functions (PDFs), describing probabilities for finding a quark in a fast-moving nucleon in a longitudinal (nucleon motion) direction carrying a fraction of the nucleon’s momentum.
In recent decades, polarised inclusive DIS experiments, using polarised lepton beams and polarised targets together with polarised proton-proton measurements, discovered that the spins of quarks and gluons contribute comparably to the spin of the proton, with a significant portion from their orbital angular momenta (OAMs). Accessing OAMs and the confined motion of the quarks inside has been a major focus for existing and future facilities.
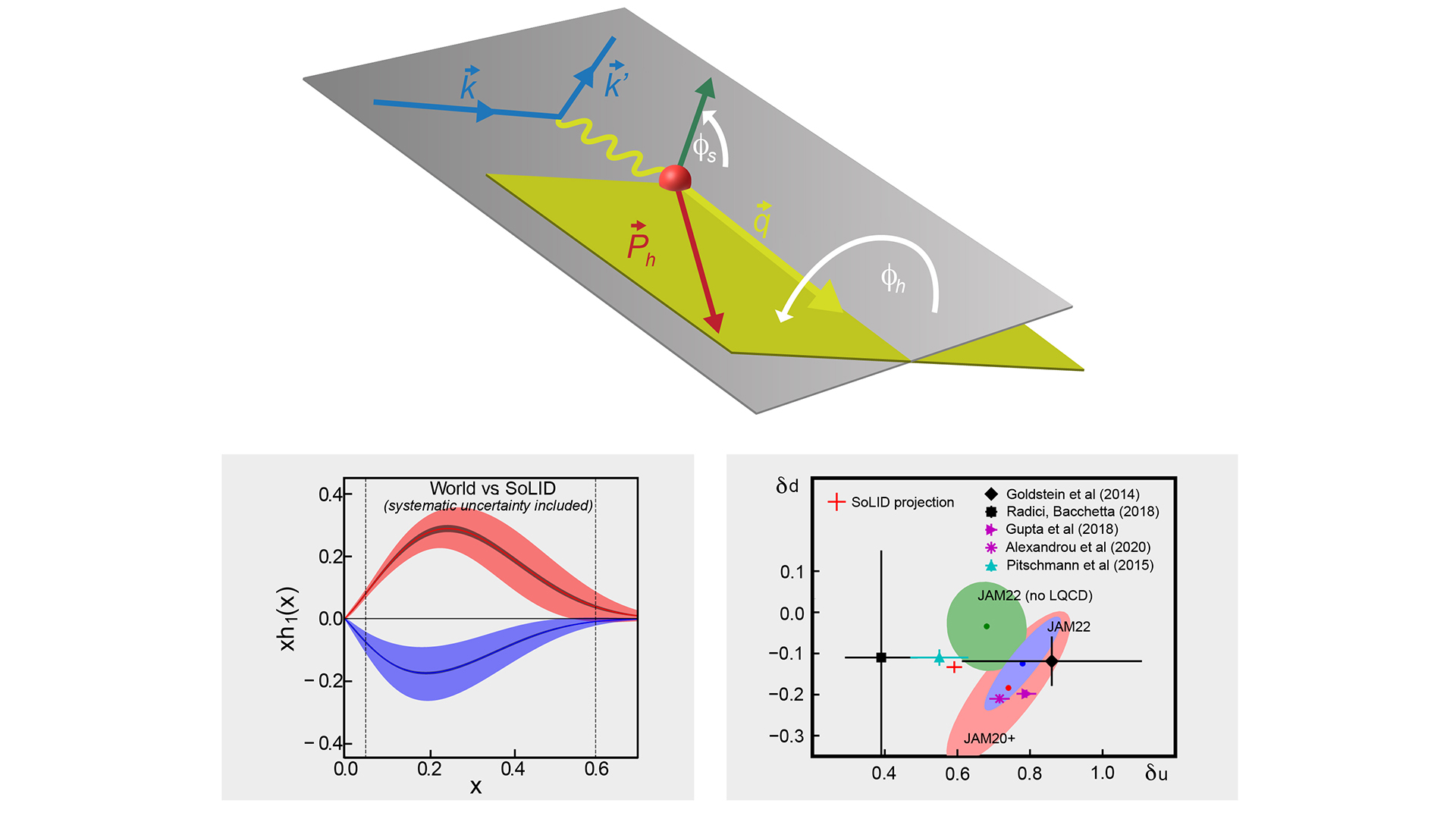
A powerful approach is through semi-inclusive DIS (SIDIS), where one detects both a scattered lepton and a leading hadron in coincidence, as illustrated in Fig. 1. SIDIS provides access to transverse momentum dependent (TMD) PDFs (TMD-PDFs, or simply TMDs), providing three-dimensional imaging of the nucleon in momentum space.
Most TMDs stem from the coupling of the quark transverse momentum to the spin of the nucleon and quark, therefore probing rich nonperturbative QCD dynamics and phenomena and providing essential information on quark orbital motion and spin-orbit correlations inside the nucleon.
The SoLID SIDIS programme2 will employ both transversely and longitudinally polarised helium-3 (3He) as a proxy for a polarised neutron and transversely polarized ammonia (NH3) targets to access TMDs in both the proton and the neutron. Such measurements will enable quark flavor separation of these TMDs. We highlight two important TMDs below.
One is the transversity TMD, which provides a direct signature for relativistic effects inside the nucleon. Its integral determines the tensor charge, an intrinsic nucleon property as important as charge or mass that has been calculated by lattice QCD. This nucleon property is also connected to the electric dipole moment of the nucleon and thus has an impact on searches for new physics beyond the standard model.3
Another is the Sivers TMD, describing a correlation between the nucleon transverse spin and the quark orbital motion. This TMD would vanish without quark OAM. With high luminosity and large acceptance, SoLID SIDIS measurements will be essential to perform the 3D momentum space imaging of the nucleon with unprecedented precision. Fig. 1 shows the impact of the SoLID SIDIS programme on the transversity TMD and the tensor charge of the u- and the d-quark. The SoLID is just as impactful on the Sivers TMD.
Proton mass, gravitational form factors, and mass and scalar radii
The electromagnetic properties of the proton have been extensively studied since Hofstadter’s pioneering electron-proton scattering experiment.4 Over the years, researchers have determined with increasing precision both the charge and the magnetic moment densities carried by the quark constituents across its size. Unfortunately, the electromagnetic probe does not directly couple to the salient gluons (strong force carriers) responsible for confining the quarks in a proton, making them difficult to explore.
Nevertheless, it is well known that gluons play a crucial role in the structure of the proton. For example, their strong colour interaction with the quarks, as well as their self-interaction, provide for a large fraction of the proton’s mass. In fact, a set of fundamental intrinsic mechanical properties, first proposed as early as the 1960s5,6 and important to characterise the proton’s structure, is still missing.
These properties are related to the proton’s energy-momentum tensor and are known as its gravitational form factors. For example, they allow one to unravel the energy density of quarks and gluons across the size of the proton, as well as describe the pressure and shear forces experienced by quarks and gluons inside the proton. Only recently have researchers been successful in providing a glimpse of these properties.7,8
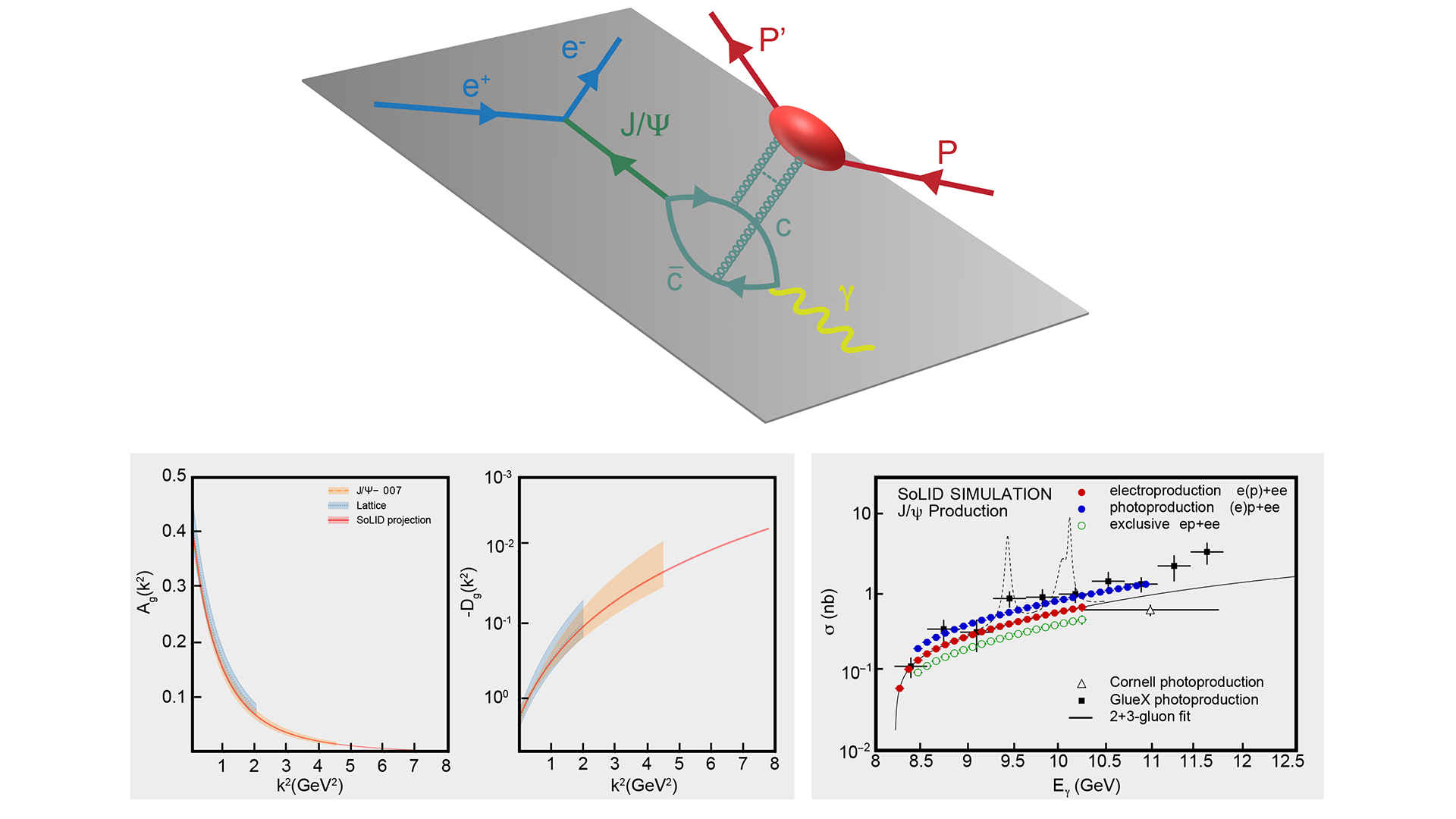
For both quarks and gluons, the first attempts at extracting these properties appear promising but will need validation across facilities in the US, from CEBAF to the Electron-Ion Collider. The preferred process to explore the gluons in the proton, as illustrated in Fig. 2, is to produce a heavy meson that then acts as a direct gluon probe in the nucleon. However, to achieve this goal, we need to operate CEBAF at high luminosities in tandem with a large acceptance detector.
SoLID at Jefferson Lab beautifully meets these requirements. Its direct impact in the determination of two critical gravitational form factors known as A and D, at an unprecedented precision, is shown in Fig. 2.
Search for physics beyond the standard model of particle physics via PVDIS
Since the late 1970s, parity violation in inclusive deep inelastic scattering (PVDIS), as illustrated in Fig. 3, of polarised electrons off unpolarised deuterium and hydrogen has emerged as a powerful tool to test the standard model of particle physics.9 It allowed researchers to confirm the electroweak theory. Over recent years, this measurement technique was enhanced to reach a level of unprecedented precision and reduced systematic uncertainty.
Today, this tool is used to explore physics beyond the standard model (BSM) through the search for small deviations in the measured asymmetries from those predicted by the standard model as well as the partonic substructure, such as the down-over-the-up quark distributions of the proton. To this end, a high statistical precision in the measurements is critical and needs to be reduced to subpercent levels, representing the most stringent requirement of luminosity and acceptance combined.
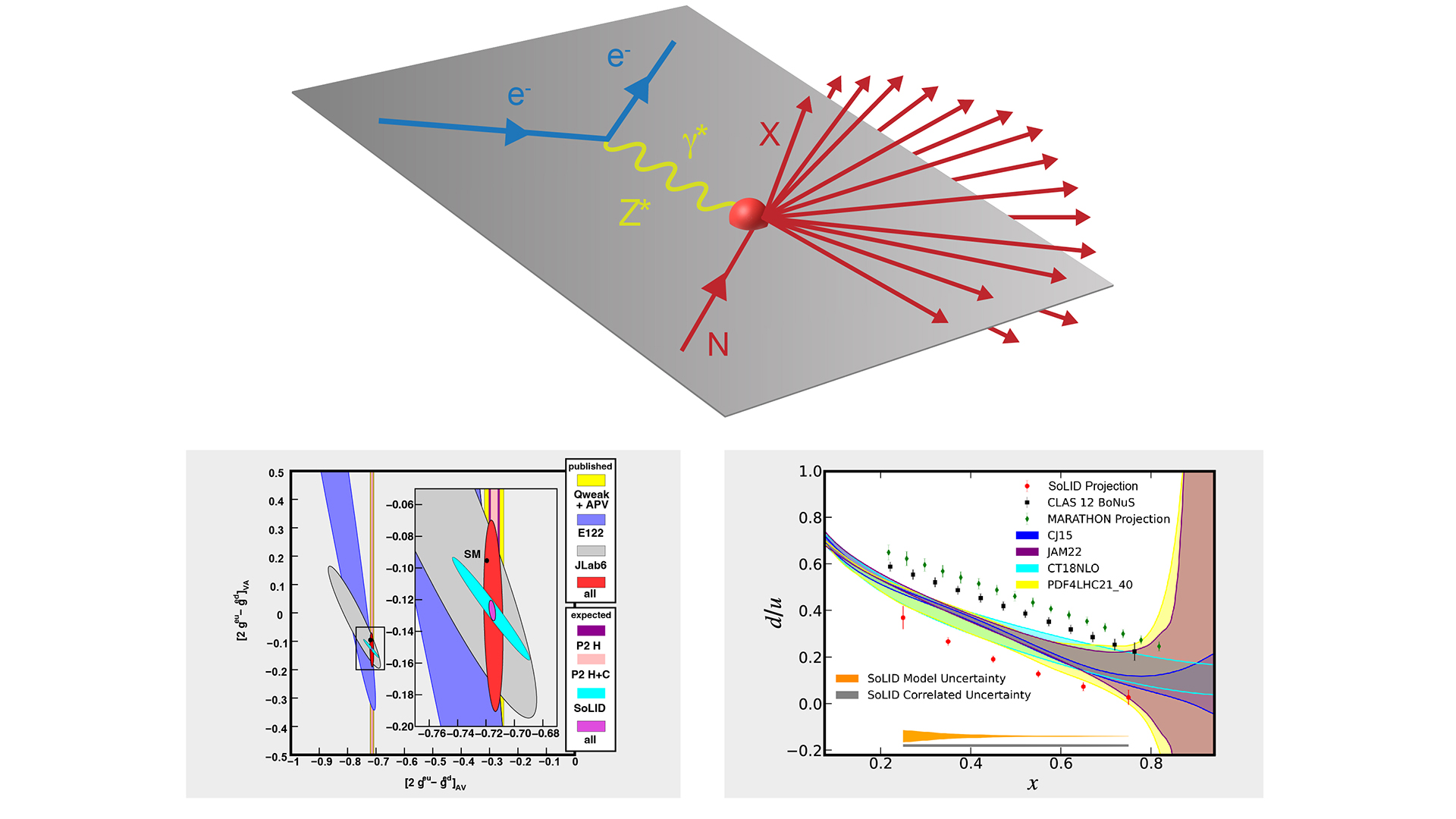
SoLID is the unique and ideal instrument allowing for the required precision in the measurement of asymmetries that should provide new constraints on the effective quark-electron (axial-vector, vector) weak couplings C2qAV to the neutral current mediated by the Z boson with a unique sensitivity to BSM like the leptophobic Z’ or dark boson Zd.
Fig. 3 shows the impact of SoLID on the standard model test and BSM search, together with the upcoming measurements of the P2 experiment10 at the MESA facility in Mainz, Germany. Also shown in Fig. 3 is the impact of the SoLID PVDIS on the PDF ratio of the d-quark over the u-quark.
Other science opportunities
The unique capability of SoLID opens the door to other exciting topics beyond the three science pillars discussed above. These include studies in SIDIS with dihadrons detected in the final state, providing a complementary approach to investigate the transversity TMD and kaons to probe the strange quarks inside
the nucleon.
A competitive programme of nucleon tomography has been developed with SoLID. Particularly promising are the most thorough studies of generalized parton distributions, enabled by SoLID using one of the most demanding processes on luminosity and acceptance, namely double deeply virtual Compton scattering (DDVCS), where both the probe photon and the emitted photon are virtual.
Most of these science topics will be studied in parallel with the approved SoLID experiments described above. Newly approved SoLID beam times will allow for studies of the two-photon exchange effect in the DIS region and the exploration of flavor dependence in the European Muon Collaboration (EMC) effect, which refers to the observed difference between the partonic structure of a free proton compared to that of a bound proton inside
a nucleus.
The SoLID apparatus, design and status
To achieve the SoLID scientific programme goals that hinge on producing high-precision results with minimal systematic uncertainty, SoLID must, as a versatile device, meet rigorous experimental requirements.
These include handling high luminosity (10³⁷ to 10³⁹ cm⁻² s⁻¹), managing high data rates, and functioning
reliably in an environment with intense radiation and significant background.
At the core of SoLID is the 1.5-tesla superconducting CLEO-II magnet, which will be modified to meet the stringent requirements for momentum and angular resolution, as well as magnetic fringe field control, essential for the use of polarised targets in the SIDIS programme.
SoLID has been designed to take advantage of modern technologies, and these include multiple layers of gas electron multipliers (GEM) as tracking detectors to achieve a resolution of 0.1 mm, a Shashlik electromagnetic calorimeter, high-performance
light and heavy gas Cherenkov detectors for particle identification, a pipeline data acquisition system (DAQ), and rapidly advancing computational capabilities including artificial intelligence and machine learning. For the PVDIS experiment, where 1039 cm-2 s-1 luminosity will be employed, baffles will be used to suppress backgrounds.
The SoLID design and its performance have been simulated and refined over the years. Recently, prototypes of all subdetectors have been built and tested on the bench and in beams. These tests,11 along with comparisons to simulations, have shown that the proposed SoLID detector, as designed, will meet the rigorous requirements of the science programme. Its readiness for construction was confirmed in a recent review,12 highlighting its potential to fully leverage the 12-GeV energy upgrade of CEBAF for the proposed high-impact science programme.
Fig. 4 shows the layout of SoLID together with its subdetectors in Experimental Hall A at Jefferson Lab.
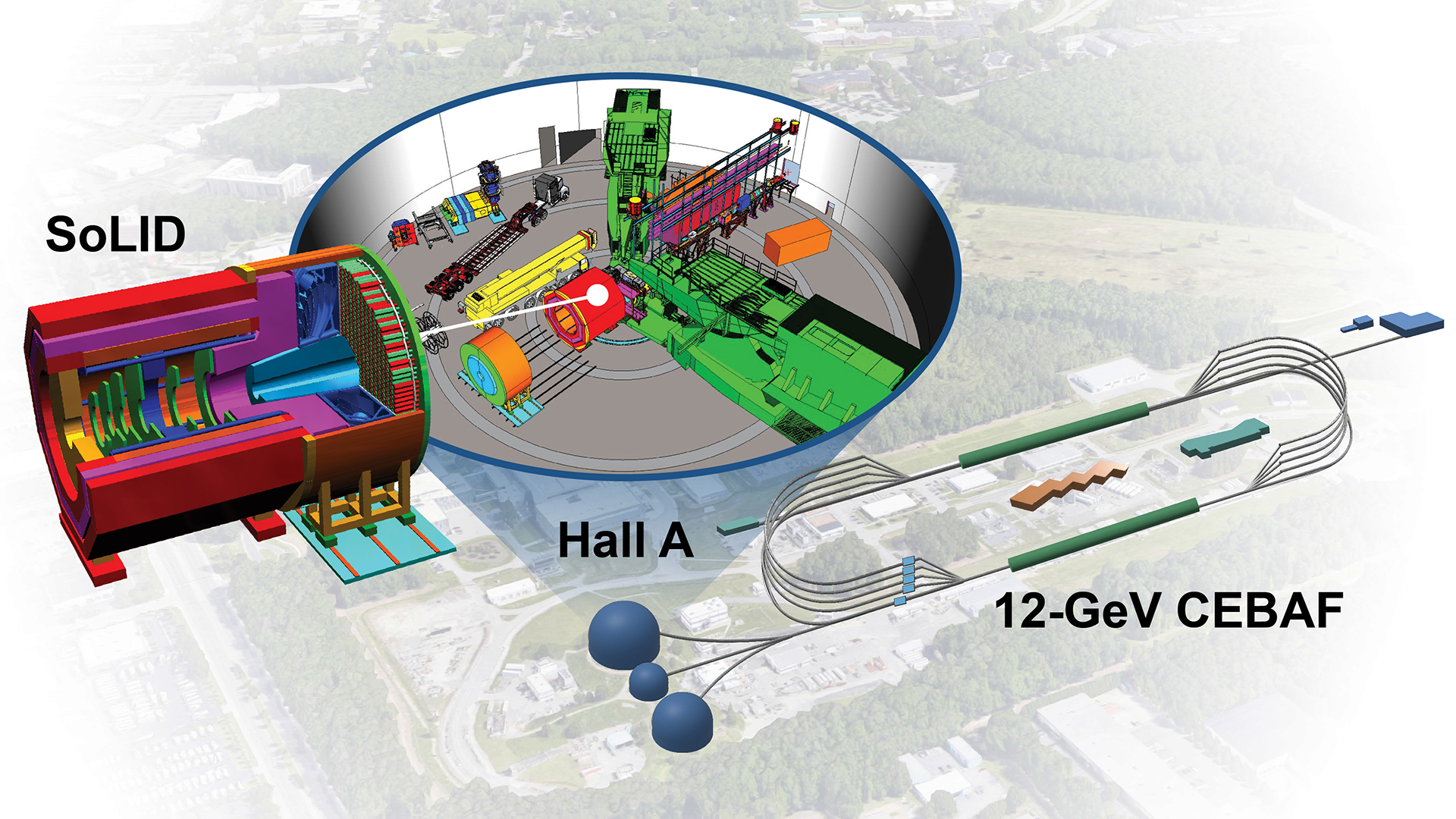
The SoLID collaboration consists of more than 270 collaborators from more than 70 institutions in 13 countries, with many theorists actively engaged and ready to unearth the science that would be revealed with the high-precision data. The SoLID science programme has received strong support from the Nuclear Physics community, as evidenced by its inclusion in both the 2015 and 2023 Long Range Plans (LRP) for Nuclear Science, led by the Nuclear Science Advisory Committee (NSAC). SoLID was highlighted in Recommendation 4 of the 2023 NSAC LRP as one of the projects that “… lay the foundation for the discovery science of tomorrow.”
Summary
Operating a large acceptance detector with a high-luminosity electron beam has long been a challenge. However, achieving this at the luminosity frontier is essential to fully harness the potential of CEBAF’s 12-GeV upgrade. SoLID, with its cutting-edge instrumentation and approved experimental programme, is a major technological leap that offers a unique opportunity to significantly expand Jefferson Lab’s scientific capabilities. It not only enhances research at the current 12-GeV beam energy but also positions CEBAF for potential higher-energy upgrades with broad scientific and societal impacts.
Acknowledgements
We thank Matthew Cahill and Joanna Griffin from Jefferson Lab for their help, along with the SoLID Collaboration. The authors acknowledge the support from Nuclear Physics, Office of Science of the U.S. Department of Energy.
References:
- J Arrington et al., Journal of Physics G: Nuclear and Particle Physics, 50, 110501 (2023)
- H Gao et al., EJPA-Plus, 126, 1-16 (2011); Z Ye et al., PLB 767, 91 (2017)
- T Liu, Z Zhao and H Gao, PRD 97, 074018 (2018)
- R Hofstadter, Rev. Mod. Phys. 28, (1956) 214-254
- I Y Kobzarev, L.B.Okun, Zh. Eksp. Teor. Fiz. 43(1962) 1904–1909.
- H Pagels, Phys. Rev. 144, (1966)
- V D Burkert, L. Elouadrhiri and F. X Girod, Nature 557, no.7705, 396-399 (2018)
- B Duran et al., Nature 615, no.7954, 813-816 (2023)
- C Y Prescott et al.Phys. Lett. B77, 347 (1978); Phys. Lett. B84, 524 (1979)
- D Becker et al.EPJA 54, 208 (2018)
- D Sharma et al.NIMA 1061, 169080 (2024); J Xie et al, JIST 19, P08011 (2024)
- https://science.osti.gov/np/nsac/Reports
Please note, this article will also appear in the 20th edition of our quarterly publication.