Professor Rene Bellwied from the University of Houston describes the latest results from heavy-ion experiments at RHIC and LHC and their relevance to our understanding of the Universe.
Over the past twenty years, the nuclear physics community has had the privilege to explore the phase diagram of matter at the two highest energy ion accelerators ever built. The parallel programmes at the Relativistic Heavy Ion Collider (RHIC) at Brookhaven National Laboratory (BNL) on Long Island, New York, USA, and the Large Hadron Collider (LHC) at CERN in Geneva, Switzerland, enable us to go back in time using heavy-ion experiments to catch a glimpse at the evolution of the Universe only microseconds after the postulated ‘Big Bang’ (see Fig. 1).
These man-made ‘little bangs’ explore the phase transition from quasi-free quarks and gluons to the particles in our present Universe and which constitute the basic building blocks of stars, planets and life itself. The quasi-free or deconfined phase of matter captures an epoch of the Universe that we were never able to access experimentally, and the creation of this so-called ‘Quark Gluon Plasma’ (QGP), even if it only exists in the laboratory for an infinitesimal fraction of a second, allows us to study two main aspects of the Standard Model of physics, namely the properties of the initial phase of matter under extreme conditions of temperature and density, and the features of the transition that shape the present day Universe and the zoo of particles it is made of.
Our group has been involved in the experimental and theoretical studies of QGP and particle formation since the beginning of the RHIC construction programme in the early 90s. We are one of the few universities that have a significant experimental programme at RHIC and the LHC. In addition, our local theory colleagues provide invaluable insight through first principle calculations by solving the theory of strong interactions – Quantum Chromodynamics – using so-called ‘lattice simulations’.
This unique synergy of experiment and theory enables us to now address many physics questions, after significantly contributing to the construction and operation of first the STAR detector at RHIC and then the ALICE experiment at the LHC. We are presently embarking on linking our discoveries not only to the question of matter formation of standard cosmological objects like the Sun or the Earth, but also to defining states of matter in compact stellar objects, such as neutron stars and black holes. In that context, the present and future results from RHIC and LHC, and their range of achievable temperatures and densities, play a significant role. This programme, which will last at least into the 2030s, will be complemented by a new electron-ion collider at the beginning of the next decade.
Heavy-ion experiments over the years
The technological advances that allow us to accelerate ions to effectively the speed of light through a subterranean circular tunnel of 27km circumference and then measure the debris of a head-on collision between two of those ions to a precision of micro-metres in distance and pico-seconds in time are unprecedented in human history. At these speeds, any particle beam on this man-made racetrack passes our stationary measuring devices more than 10,000 times in a single second.
It took ten years to build RHIC and then another ten to complete the LHC. By making use of multi-layered detectors the size of football fields and the weight of the Eiffel tower, we capture the formation and development of the ‘Little Bang’ to the highest precision. It takes not only collaborations of thousands of highly trained physicists from more than 200 countries to record, analyse, and interpret the data, but also the international co-operation of many governments to provide the funding to build, operate, and maintain these machines and experiments over their lifetime of about 30-40 years. This is a global human effort in its truest sense, collaborative without borders for the greater good and a deep insight into our existence.
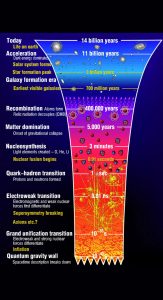
How to create matter out of energy
The idea of creating visible matter out of energy early in the evolution of the Universe is one of the cornerstones of the Standard Model of modern physics. In essence, this process can be broken down into two specific steps. First, the inner building blocks of the matter we know, i.e. the quarks, attain their mass by interacting with the Higgs field, whose force carrier, the Higgs boson, was discovered at the LHC in 2012 and led to the Physics Nobel Prize in 2013. Second, the quarks and the carriers of the strong force, the gluons, interact and form confined objects, such as protons and neutrons. It is this second step, which is important to understand the present state of the Universe.
In order to study the interactions and confinement of quarks and gluons, one has to first deconfine them through a microscopic collision of highly accelerated ions the size of a few femto-metres, which generates an energy density that is equivalent to the annual energy consumption of the United States compressed into a 100μm3 box.
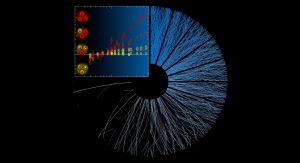
A strange new world
The discovery of the QGP was first announced in 2005, jointly by the four heavy-ion experiments, based on evidence from the flow of matter after the collision and the energy loss of particles in the created medium, which could be attributed only to quark contributions from a deconfined system.1
There had been indications of a QGP a decade earlier from experiments at CERN, which at the time had measured unique patterns of particle production attributed to the enhancement of certain heavier particles, containing strange quarks, and the suppression of others, containing charm quarks. All visible stable matter in the Universe is presently made of only the two lightest quarks, the up and down quarks. Although production of heavier quarks is not per se a signal of deconfinement, their abundance relative to the light quarks is crucial in defining unambiguous signatures. These early measurements were revived at the LHC in a much more differential way, which indeed proved that strangeness enhancement is a key signature down to the smallest available collision systems as shown in Fig. 2. That means that even collisions between two protons might form a plasma-like state.2
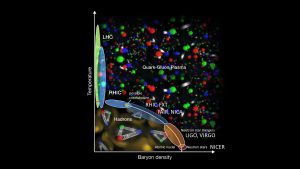
Antimatter and exotica
One of the big open questions of modern physics has been solved through the balanced formation of quarks and anti-quarks in the QGP. Scientists could never prove whether antimatter can form in the Universe just like matter, as long as the anti-matter is isolated from the annihilation process it undergoes when it comes in contact with matter. Since the QGP is an anti-quark factory, experiments at RHIC and LHC were able to isolate and characterise nuclei, up to anti-helium, completely made of anti-particles.3 These studies were also confirmed more recently by antimatter measurements in space using the AMS satellite.
Another major discovery from this formation of matter epoch is evidence from the LHC experiments that not only particles made out of three quarks or one quark-antiquark pair are possible in Nature, but so are tetra-, penta- and maybe even hexa-quark states. Although not forbidden by the Standard Model of physics, these states were previously never experimentally verified. Until now, all these multi-quark configurations required the inclusion of a very heavy quark, the charm quark, which is not well understood at this time. Based on the enhanced number of strange quarks in the produced heavy ion system, there is also an ongoing search for these states in the strange sector.
In summary, the matter zoo has been significantly expanded based on the first ten years of LHC operations, and the question arises as to how these measurements impact our deeper understanding of the Universe and its evolution.
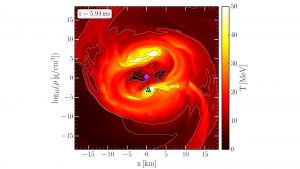
A critical point in the phase diagram
One of the open questions that remain is the evolution of matter as a function of increasing density and decreasing temperature. The commonly accepted phase diagram is shown in Fig. 3. It should be noted that the diagram is bracketed on the high temperature, low density side by the early Universe estimates, which are achievable at the high energy accelerator facilities, and on the low temperature, high density side by compact stellar objects in the present Universe, namely neutron stars. In between, it is believed that the phase transition changes its order from a cross-over behaviour to a first order phase transition.
This would require the existence of a critical point, not unlike the one seen in water at high pressure and temperature. In order to achieve these experimental conditions in high energy particle collisions, one actually has to decrease the collision energy. Rather than the two ions crashing through each other, in this case the ions would stop and thus generate a system of very high density and relatively low temperature.
With the onset of the highest energy collisions at LHC in 2010, RHIC decided to embark on a programme of lower energies in order to map out any evidence for critical behaviour. A critical point leads to many measurable effects inside a defined region of temperature and density. The change from a smooth transition at low density to a discontinuous first order transition at higher density is accompanied by a sudden release of energy and many observables, such as the number of produced particles, should start to critically fluctuate. The first round of experiments has already yielded tantalising evidence for such behaviour, but in order to make an unambiguous statement, more collisions are needed, and these are presently scheduled at RHIC for 2020-2022.
The fluctuation measurements also revealed that, from the highest energies at the LHC down to the critical region in the phase diagram, there might be a quark flavour dependence on the temperature at which particles are formed out of the QGP. In other words, particles with heavier quark constituents form earlier than protons and neutrons that are made from light quarks. These findings at high temperature might relate to another puzzle at the lowest temperature and highest density, namely the matter in the core of neutron stars.
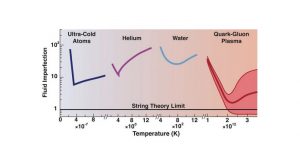
From the early Universe to the densest stars
Scientists have long hypothesised that quark matter exists in the core of neutron stars. This could explain the very large mass (up to two times the mass of the Sun) in a very small object (a few tens of km radius). The creation of an over-abundance of strange quarks, plus their potential decoupling from a plasma phase at a higher temperature, opens up the possibility of creating ‘strange matter’, i.e. rare states of quark configurations which are dominated by strangeness.
Our experiments have already discovered the formation of hyper-nuclei, i.e. nuclei in which one of the common protons or neutrons is replaced by a similar particle with strange content. These composite objects are meta-stable, and, surprisingly¸ so are their anti-nucleus partners. This discovery, together with the new measurements of attractive forces between light quark and strange quark particles, leads some of us to believe that strange matter, i.e. matter in which the strange quark occupies the space normally reserved for light quarks in ‘standard nuclear matter’, could exist and form the core of many compact stellar objects, whose unusual mass-to-size ratio is otherwise not well understood.
One avenue of cross-disciplinary research arose from the ground-breaking discovery of gravitational waves, generated by the merger of two black holes. This 2017 Nobel Prize winning measurement has been followed up by the LIGO and VIRGO experiments at a higher precision scale to detect the merger of two neutron stars, i.e. much smaller, but still very dense objects.
Neutron stars are relatively easy to characterise compared to the rather elusive black holes. Their structure, although tiny in size in the vast dimensions of the Universe, can be modelled and separated into several phases of matter as a function of the star radius. The link to relativistic heavy ion physics comes through the dynamic modelling of the core of the merging matter, which is likely a plasma and possibly strangeness enhanced state at temperatures and densities comparable to low energy heavy ion collisions (see Fig. 4).
Neutron star mergers leave a distinct gravitational wave spectrum and the analysis of this spectrum might allow us to determine the phase and potential phase transitions during the merger process, which would map out a uniquely different part of the QCD phase diagram, namely the low temperature, high density part (see Fig. 3).
How unique is the QGP?
Finally, the properties of the deconfined quark-gluon matter are often defined through the collective motion of this early phase after the collision. So called ‘flow’ measurements have shown that the system behaves like a near-perfect liquid, which means the shear viscosity over entropy ratio, a measure of the fluid imperfection, is minimal and significantly lower than the one for ultra-cold liquid helium, which was considered the most perfect experimentally achievable liquid until now (see Fig. 5).
This perfect liquidity is a sign for very strong interactions between the degrees of freedom in the plasma, similar to string theory calculations of interactions in black holes. The analogy between theoretical black hole physics and experimentally verifiable heavy ion collisions led to an interesting cross-disciplinary field of applying gravitationally motivated equations to strong force coupled systems. For example, additional state variables such as bulk viscosity, speed of sound, and heat capacity can be derived from existing measurements and serve as experimental input to an equation of state, which at the same time can be derived from theoretical first principle calculations as long as the matter density in the system is small. For high densities, though, the lattice QCD calculations need to be extrapolated by, for example, using black hole inspired model analogues. Nevertheless, the predictions for a critical point near the energies achievable at existing accelerators and calculations beyond this point into the regime of neutron stars are at hand, and their experimental verification awaits us in the next two decades.
The future and quantum entanglement
The path forward for heavy-ion experiments is well defined through long range plans that are drafted by the expert community under the guidance of the funding agencies in Europe, Asia, and the United States. The LHC heavy ion programme will continue for at least another decade. The RHIC programme will wind down and make room for an electron-ion collider (EIC), scheduled to come online in the early part of the next decade. The goals, to be addressed by colliding a structure-less elementary particle, such as the electron, with a proton or a heavy ion, is to study even more aspects of the structure of matter.
One, which is of particular interest to our group, is the role of quantum entanglement in a collision of particles composed of quarks and gluons. Quantum-mechanically, these fundamental building blocks know of each other within a confined space and thus share certain properties, which should affect the distribution of particles emerging from the plasma state. Until now, it was computationally too difficult to apply quantum mechanics to the QGP, but with the onset of quantum computing and a new accelerator that probes the inner structure of nuclear matter with an indivisible probe, such as the electron, we should be able to study entanglement in high temperature and density systems governing the earliest phases of the Universe. This is indeed an exciting time to be a fundamental physicist.
Acknowledgements
This work was supported in part by the Office of Nuclear Physics of the U.S. Department of Energy.
References
- ‘The RHIC Collaboration’s critical assessment of QGP evidence from RHIC collisions’, Nucl.Phys.A 757 (2005) 1
- ‘Enhanced production of multi-strange hadrons in high-multiplicity proton-proton collisions’, J. Adam et al. (ALICE Coll.), Nature Phys. 13 (2017) 535
- ‘Observation of the antimatter helium-4 nucleus’, H. Agakishiev et al. (STAR Coll.), Nature 473 (2011) 353
- ‘Neutron Star Mergers: Probing the EoS of Hot, Dense Matter by Gravitational Waves’, M. Hanauske et al., Particles 2 (2019) 1, 44
- Figure taken from ‘Implementing the 2007 Long Range Plan’, Report to Nuclear Science Advisory Committee at https://science.osti.gov/np/nsac
Professor Rene Bellwied
MD Anderson Professor of Physics
Physics Department
University of Houston
+1 713 743 3548
bellwied@uh.edu
https://sites.google.com/nsm.uh.edu/rene-bellwied
Please note, this article will also appear in the third edition of our new quarterly publication.