The Leprince-Ringuet Laboratory (LLR) explores the interplay between particle physics and cosmology, with high energy and neutrino physics experiments as well as with telescope arrays and satellites for gamma astronomy.
The Leprince-Ringuet Laboratory (LLR) is a particle physics laboratory of the French National Institute of Nuclear and Particle Physics (IN2P3). The laboratory is under the joint supervision of the French National Center for Scientific Research (CNRS) and the École Polytechnique. The LLR was founded in 1936 by Louis Leprince-Ringuet as the very first research laboratory of the École Polytechnique and was devoted from the beginning to the study of atomic nuclei, cosmic rays, and the knowledge of fundamental particle physics. Its actual research programme covers particle and heavy ion physics at large colliders, gamma (γ) astronomy, neutrino physics, the development of new plasma acceleration techniques, and detectors for applications in hadron beams for cancer therapy.
Fermi Large Area Telescope
In high energy γ-ray astronomy, the LLR has contributed to the building of the Large Area Telescope (LAT) of the Fermi satellite, which was launched back in June 2008 and continues to provide essential data for particle physics experiments and γ rays in the 50 MeV to 300 GeV high energy range. The laboratory oversaw the mechanics of the calorimeter, one of the three subsystems of the instrument. It is made of 16 modules, each containing 96 bars of caesium iodide (CsI) maintained in light alveoli made of composite material. Less than a year after its launch, Fermi-LAT had already discovered more than a dozen new pulsars, the residual core of a massive star after its supernova explosion, emitting only in the γ-ray range. The harvest of data continues today with more than 5,000 γ-ray sources in the latest catalogue, and Fermi has recently observed events coinciding with the detection of gravitational waves by the interferometers LIGO and VIRGO. In autumn 2022, Fermi detected the brightest gamma-ray burst in the history of observations (GRB 221009A), with the highest total isotropic-equivalent energy ever observed (1 × 1055 erg) and second-highest isotropic-equivalent luminosity (9,9 × 1053 erg/s).
Above the energy range of Fermi-LAT, the flux from the most intense γ-ray sources become so small that instruments with very large effective area (> 105 m²) are needed, which can only be built on the ground and rely on the development of atmospheric showers. The bestiary of transient violent events in the Universe has been considerably enlarged thanks to the array of Imaging Atmospheric Telescopes H.E.S.S., which studies cosmic gamma rays in the energy range from 100 GeV to about 100 TeV (VHE), complementary to that of Fermi-LAT. The LLR designed and manufactured the mechanics of the cameras for the four 13m diameter telescopes, as well as the camera for the large 28m diameter telescope (CT5) installed in Namibia in September 2012. The H.E.S.S. array has allowed the detection of very faint particle physics sources, such as the first starburst galaxy (NGC 253) or the supernova remnant (SN 1006). Recently, the H.E.S.S. group at the LLR has contributed to the observation of a record acceleration of cosmic particles in a recurrent nova. Such transient phenomena correspond to repeating thermonuclear explosions situated in the outer layers of white dwarfs and caused by the accretion of matter from a binary companion. Particles get accelerated by the shockwave, which is generated when the ejected material crashes into the companion star’s wind.
Thanks to Fermi-LAT and H.E.S.S. and to other observatories, the observation of violent transient phenomena in the Universe has undergone a revolution in the last 20 years with the observation of thousands of new sources of high energy cosmic rays, from pulsars to pulsar wind nebulae, supernova remnants, active galactic nuclei (supermassive black holes that emit bright jets and winds), binary systems, etc.
The LLR is now involved in the construction of instruments for the next generation of telescopes for VHE gamma-ray astronomy with the CTA project. The objective is to improve the sensitivity by a factor of five to ten in the energy range between 100 GeV and 10 TeV. The laboratory is involved in the design and production of the NectarCAM camera that will be used for particle physics experiments on medium-size telescopes (MSTs).
CMS experiment at the Large Hadron Collider
In particle physics at high energy colliders, the fundamental interactions at very short distances reproduce the conditions of the very early Universe. The LLR has contributed to the construction of the Compact Muon Solenoid (CMS) experiment at the CERN Large Hadron Collider (LHC) near Geneva and designed and built the trigger system for the electromagnetic calorimeter composed of lead tungstate crystals.
The CMS group at the LLR was among the protagonists of the discovery of the Higgs boson in 2012. The Higgs boson (spin 0) is associated with the Higgs quantum field responsible for the Brout-Englert-Higgs (BEH) spontaneous electroweak symmetry breaking (EWSB) mechanism and the appearance of the mass of elementary particles in the very early Universe. The phase transition is presumed to have happened towards the end of the inflation phase of space-time, when the temperature dropped below a critical value, about 10-12s after the Big Bang. Since that decisive moment after the creation of the Universe, the nature of the quantum real vacuum has been frozen, and matter particles (spin ½), as well as fundamental interactions carried by vector bosons (spin 1), appear as we know them today. The electromagnetic interaction has an infinite range, as it proceeds via the exchange of zero mass photons between two electrically charged fermions. The weak interaction is very short-range because of the high masses of the Z° (91 GeV) and W± (80 GeV) bosons acquired through the BEH mechanism. Moreover, it is a Yukawa coupling with the Higgs quantum field pervading the whole Universe that is responsible for the appearance of the mass of elementary fermions. Once the mass of the discovered Higgs boson is fixed at 125 GeV, the particle physics theory becomes extraordinarily constrained. Following the discovery of the Higgs boson, analyses of new data from the ATLAS and CMS experiments at the LHC confirmed the existence of Yukawa interactions and showed that the couplings to the vector bosons and elementary fermions were well within theoretical predictions.
The shape of the Higgs field potential responsible for the BEH mechanism depends on the Higgs boson’s trilinear self-coupling, i.e. the way the Higgs boson couples with itself. In practice, this means being able to access H-boson pair production. This will become possible by pushing the performance of CERN’s Large Hadron Collider to its maximum during a new phase of very high luminosity operation, called the High-Luminosity Large Hadron Collider (HL-LHC), scheduled for 2029 onward. The LLR laboratory is involved in the development of the mechanics and triggering electronics of a new type of high-granularity calorimeter (HGCAL) for the CMS particle physics experiment at HL-LHC. The aim is to build two identical detectors to replace the current end caps (see photo below), closing the front and rear ends of the cylinder formed by the experiment, and which will have to survive an extremely hostile environment, with integrated radiation doses of up to 2 MGy and a fluence of 1016 neutrons per cm2.
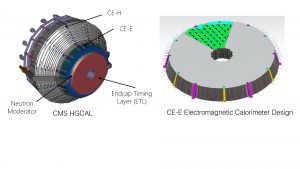
The detectors must also be able to cope with a proton beam crossing frequency of 40 MHz while sorting out the hundreds of collisions that will occur at each crossing. The solution adopted is necessarily particularly complex, and so is the HGCAL, with readout planes made of silicon tiles on tungsten bases and lead absorber planes. The granularity of this new calorimeter is unprecedented in high-energy physics, with more than six million readout channels per end cap for 0.5 and 1.0cm2 silicon cells, all feeding analogue signals to state-of-the-art electronic chips (HGCROC) developed by the OMEGA microelectronics centre, an IN2P3 research platform adjacent to the LLR at the École Polytechnique. The HGCAL imposes extremely challenging specifications for the front-end electronics, such as high dynamic range, low noise, high precision timing information and low power consumption, while simultaneously selecting and transmitting trigger information.
Production tests of these front-end readout ASICs will be performed with new robotic platforms built at the LLR and installed both at LLR and OMEGA. The HGCAL will provide a complete reconstruction of the energy, momentum, and time of flight of the different particles produced at the collision point. It will be a key element in the reconstruction of the flux of particles created by each collision, with a major impact on all particle physics analyses at HL-LHC.
Besides electroweak and strong nuclear interaction physics in CMS, the LLR is also strongly involved in the analysis of heavy ion collision data from the CMS and Large Hadron Collider beauty (LHCb) experiments for the study of a new state of hadronic matter called the quark-gluon plasma which existed in the first few microseconds after the Big Bang.
T2K neutrino experiment
The LLR joined the Tokai to Kamioka (T2K) neutrino physics experiment in Japan more than 15 years ago. One of the main motivations is the search for CP violations in the neutrino sector. CP violation is an essential prerequisite for explaining the matter-antimatter asymmetry in the Universe. The T2K experiment is a so-called long-baseline experiment exploiting beams of muonic neutrinos produced by the JPARC accelerator situated in Tokai. To study neutrino oscillations, the particles produced at JPARC are directed to the Super-Kamiokande (SK) far-field detector located 295km downstream. A set of nearby detectors is used to estimate the number of interactions expected in SK in the absence of oscillations. The LLR has strongly contributed to the construction of the INGRID near detector with the design of the mechanics and electronics.
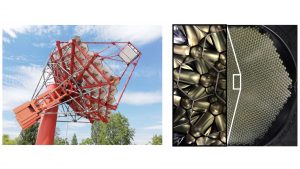
It is currently involved in the realisation of the readout electronics for the upgraded super Fine-Grained Detectors (sFGD) of ND280. In order to obtain better control of systematic uncertainties due to the limited knowledge of the neutrino-nucleus interaction cross sections and the difference between the ND280 (plastic) and SK (water) detectors, the LLR has furthermore been involved in the mechanical design, simulations and development of the acquisition chain of a dedicated Water Grid and Scintillator (WAGASCI) experiment. The T2K particle physics experiment announced the first observation of the vμ→ve oscillation in 2013 and a first indication for CP-violation in the neutrino sector in 2020, with CP conservation ruled out at 90% confidence level. The particle physics experiment (T2K-II) is scheduled to resume taking data with the upgraded ND280 detector towards the end of 2023.
Diffuse Supernova Neutrino Background
The LLR, moreover, joined the SK experiment in 2016 and is involved in particular in the cosmology-oriented search for the Diffuse Supernova Neutrino Background (DSNB). The DSNB is composed of neutrinos of all past core-collapse supernovae (SN). These are among the most cataclysmic phenomena in the Universe and important ingredients of the dynamics of the cosmos, with 99% of the energy released in the form of neutrinos, and up to 1058 neutrinos emitted in a single SN burst. The DSNB could provide information not only on the supernova emission process but also on star formation and the history of the Universe’s expansion. The signal from DSNB neutrinos is to be provided by inverse beta decay reactions (ve + p →e+ +n) in the detector and the main challenge concerns the identification of the neutron capture. From 2020 onwards, the SK water detector has been doped with Gadolinium sulfate, which considerably improves the signal of a neutron by giving rise at capture to a cascade of photons with total energy summing to ~ 8 MeV. Finally, the LLR is involved in the preparation of the Hyper-Kamiokande (HK) experiment, which will have a fiducial volume of water approximately ten times larger than that of SK. This has provided the LLR neutrino group with an opportunity to develop with OMEGA a new digitiser chip called HKROC, which evolved from the structure and concepts of HGCROC, and which would fit the requirements of a neutrino physics experiment equipped with photomultiplier tubes such as HK.
Please note, this article will also appear in the fourteenth edition of our quarterly publication.