Professor Carsten Rott discusses exploring the Universe with high-energy neutrinos to identify the sources of the high-energy cosmic rays and in search for new phenomena.
Most of what we know about the Universe originates from observations of electromagnetic radiation from stars, planets, distant galaxies, or other such astronomical objects. In a quest to gain a deeper understanding of these objects, astronomical observations have expanded from initially just covering the optical band to an ever-broader wavelength spectrum. Nowadays observations cover the entire range from radio waves to gamma-rays.
Particles generated in stellar explosions, or in the vicinity of black holes, can carry energies millions of times higher than those produced in the most powerful particle accelerators on Earth. Observing such particles not only allows us to directly infer the processes that might occur to generate them, but whether they are also susceptible to new phenomena, which could occur above the energy scales that have been experimentally tested in our laboratories.
At highest energies, the universe is impenetrable to electromagnetic radiation. Very high-energy cosmic rays share a similar fate. They interact resonantly with the cosmic microwave background, making it impossible for any particles to reach us from distant sources. Fortunately, neutrinos, the most elusive particles found in the standard model of particle physics, provide just the right characteristics to explore the distant universe and the most extreme environments found in space.
The cosmic sources responsible for accelerating particles to extreme energies are expected to also generate high-energy neutrinos. This close link enables us to solve the century old problem of the identification of the unknown sources of high-energy cosmic rays via the detection of neutrinos. Neutrinos do not carry any electric charge, which means they will not be deflected by magnetic fields and their trajectories point back to their astrophysical birthplaces. Neutrinos are weakly interacting particles, this property makes them elusive, but at the same time allows them to escape from dense stellar environments that are otherwise opaque to radiation.
State of the field
Astroparticle physics has emerged as a core scientific discipline that harbors tremendous discovery potential. Its importance is marked by a large share of Nobel prizes related to this field over the last few decades. Following the discoveries of gravitational waves and high-energy astrophysical neutrinos, the field is in the midst of a revolution. The IceCube neutrino observatory has been at the forefront of exploring the Universe with high-energy neutrinos. Recent scientific breakthroughs by this observatory, combined with a diverse high-impact science programme have triggered a broad interest in neutrino telescope science. International efforts are underway to construct new telescopes in Lake Baikal (Baikal-GVD), the Mediterranean Sea (KM3NeT), the Pacific Ocean (P-ONE), and to further enhance the IceCube observatory at the South Pole. Similarly, large-volume underground neutrino detectors are under construction in Japan (Hyper-Kamiokande), the US (DUNE), and a detector in Korea is under intense discussion (KNO).
The IceCube Neutrino Telescope
The IceCube Neutrino Observatory is the world’s largest neutrino telescope and is located near the South Pole. The observatory consists of a surface air shower array and detector units buried 1500m to 2500m below the surface of the ice. More than 5000 detector units, called digital optical modules (DOMs), are distributed over a cubic kilometer of ultra-pure Antarctic ice. Faint flashes of Cherenkov light are produced when neutrinos interact in the ice. The IceCube Neutrino Observatory has been constructed over a period of seven austral summer seasons. Since its completion in 2010, it has executed a highly successful scientific programme over a broad science scope ranging from the ground-breaking observations of high-energy astrophysical neutrinos to measurements of the particle properties of neutrinos and leading bounds on dark matter properties. Competitive measurements of neutrino properties, atmospheric and astrophysical neutrino measurements are made possible by IceCube’s capability to detect neutrinos with energies from 10 GeV to beyond 109 GeV, as well as bursts of neutrinos generated by stellar explosions.
Astrophysical Neutrino Sources and Flux
Following the observation of a diffuse astrophysical neutrino flux by the IceCube Neutrino Telescope in 2013, the hunt is now on to identify the sources of the high-energy neutrinos. On 22 September 2017, IceCube detected a very energetic neutrino coming from a region of the sky that contained an active galaxy with a supermassive black hole at its centre. The black hole gives rise to a jet of ultra-relativistic particles that happens to point directly towards the Earth. There are thousands of these objects, called blazars, all over the sky, but what made this event so special is that the Fermi-LAT space telescope and the MAGIC gamma-ray telescope in La Palma, Spain, recorded gamma rays with high energies from the same region on the sky at the same time. The chance of such a coincidence was found to be around one in a thousand. Following this evidence, IceCube searched through archival data and found that more than a dozen neutrinos were detected from the direction of the blazar between 2014 and 2015. This rate is unusually high compared to expectations from neutrinos generated naturally in the Earth’s atmosphere. This makes the likelihood of the neutrinos originating from the blazar, known as TXS0506+056, with a distance of 4 billion light-years, also about one in one thousand. Very high.
Search for new Phenomena with Neutrinos
With the observation of high-energy extra-terrestrial neutrinos, a new era in astroparticle physics has begun. We have observed neutrinos with unprecedented energies, coming from the depth of the Universe, further than any previous measurements. Observing these neutrinos not only pushes forward our understanding of the cosmic accelerators, but also opens doors to study new phenomena. Very rare processes occurring on the long journeys of the neutrinos from their sources can be tested that are beyond the reach of anthropogenic neutrino beams. Energy scales above the reach of accelerators can be explored to test for signs of physics beyond the standard model of particle physics.
Indirect Search for Dark Matter with Neutrinos
There is compelling observational evidence for the existence of dark matter from numerous independent measurements. Despite the overwhelming case for the existence of dark matter, the underlying nature of this mysterious matter remains unknown. Most attractive have been models that solve fundamental problems in particle physics and naturally yield dark matter particle candidates. The hunt for particle dark matter candidates is pursued with direct searches, indirect searches, and by using particle colliders that recreate the extreme environments, that existed in the early universe when dark matter particles would have been created.
Some of the most stringent bounds on the interaction rate of dark matter with ordinary (baryonic) matter have been obtained by looking for energetic neutrinos from the Sun. In this scenario, dark matter from the Galactic dark matter halo, might become trapped in the centre of the Sun. If a dark matter particle loses enough momentum by scattering off a proton in the Sun, such that its velocity falls below the escape velocity of the celestial body, the particle will be gravitationally trapped. As dark matter particles accumulate in the centre of the Sun, they may eventually self-annihilate with each other resulting in the steady production of standard model particles, of which only neutrinos can escape the dense solar environment. Despite the seemingly complex process of dark matter capture in the Sun, the expected neutrino flux generated by dark matter annihilations in the centre of the Sun is very well understood and extremely robust against changes in underlying conditions. This makes experimental limits on dark matter properties minimally dependent on uncertainties in the dark matter velocity distribution, or variations in the dark matter density in the vicinity of the solar system.
Indirect evidence for dark matter might also be observable by studying the arrival direction and energy spectrum of astrophysical neutrinos. Decaying or self-annihilating dark matter models predict characteristic distributions of neutrino events over the sky following that of the Galactic dark matter halo density. Searches using IceCube data have been carried out and have proven to be extremely sensitive to heavy decaying dark matter. No excess of events was observed and some of the most stringent bounds on the dark matter lifetime were set, in some cases exceeding the age of the Universe by a factor of 100 billion.
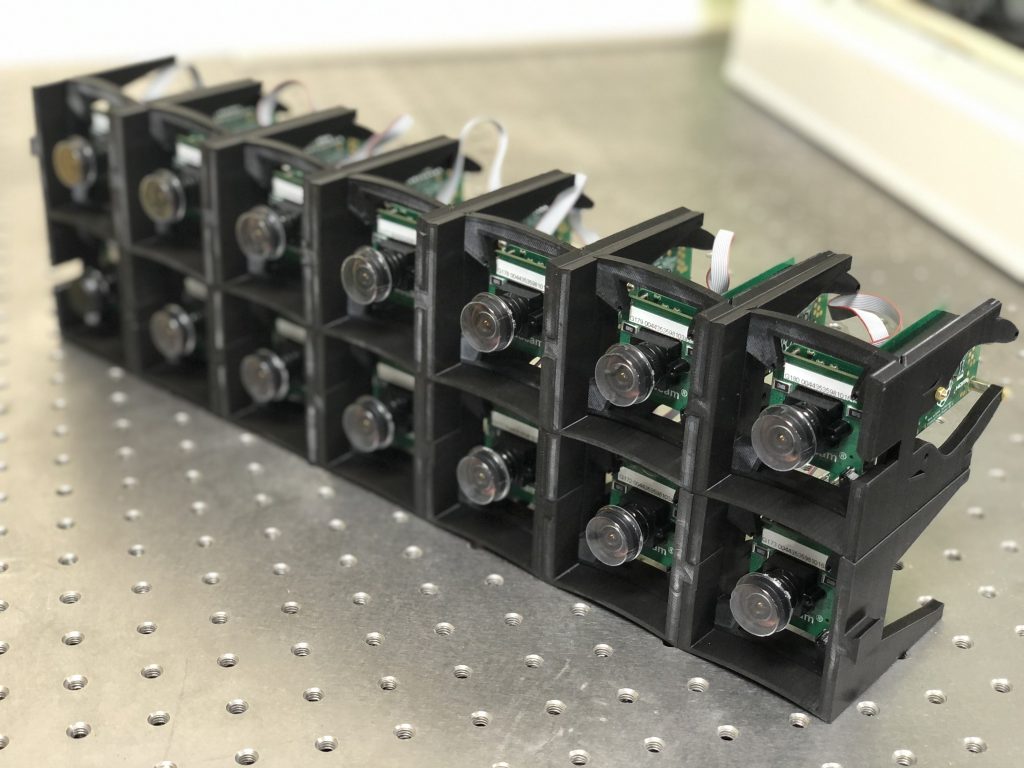
The IceCube Upgrade
An upgrade to the IceCube Neutrino Observatory is currently under construction. For the upgrade, seven new strings will be deployed in the central region of the 86 string IceCube detector to enhance the capability to detect neutrinos in the GeV range. The main scientific objective of the upgrade is to carry out precision neutrino oscillation studies and to search for new physics that might be apparent through the non-unitarity of the neutrino mixing matrix. A further objective of the upgrade is an improved calibration of the IceCube detector to reduce uncertainties related to the optical properties of the Antarctic ice. Reliable neutrino event reconstruction to determine neutrino energies, arrival directions, or flavour types rely on the capability to precisely model light propagation in the Antarctic ice. While the IceCube collaboration has acquired a remarkable understanding of the optical ice properties that even includes anisotropy in the light propagation in the ice, additional calibration studies are expected to further improve neutrino event reconstructions.
For the IceCube upgrade, more than 2000 cameras and illumination systems will be deployed to more precisely measure photon arrival directions from distant light sources. The novel camera-based calibration system will be used to measure photon arrival directions and to survey properties of the refrozen ice in drill holes. The upgrade is currently planned for deployment in 2022-2023 season, the schedule is under review due to the impacts of the COVID-19 pandemic.
IceCube-Gen2
IceCube observations have allowed us to discover a diffuse flux of high-energy neutrinos of extra-galactic origin. The data has further provided us with the first evidence on the sites of cosmic ray acceleration outside of our Milky Way Galaxy. The astrophysical neutrino spectrum and the candidate sources observed so far suggested that the neutrino sky is complex with multiple different types of cosmic accelerators wielding the neutrino flux.
IceCube-Gen2 foresees the construction of an approximately ten times larger detector that will be able to explore the neutrino sky with sufficient statistics to make conclusive statements about astrophysical neutrino sources and search for hints of new physics. The in-ice optical array will be complemented by a surface air shower array and an extended radio detector array. IceCube-Gen2 will open up a new frontier for discoveries as the accessible sample size increases significantly. The observatory will play an essential role in shaping the new era of multi-messenger astronomy.
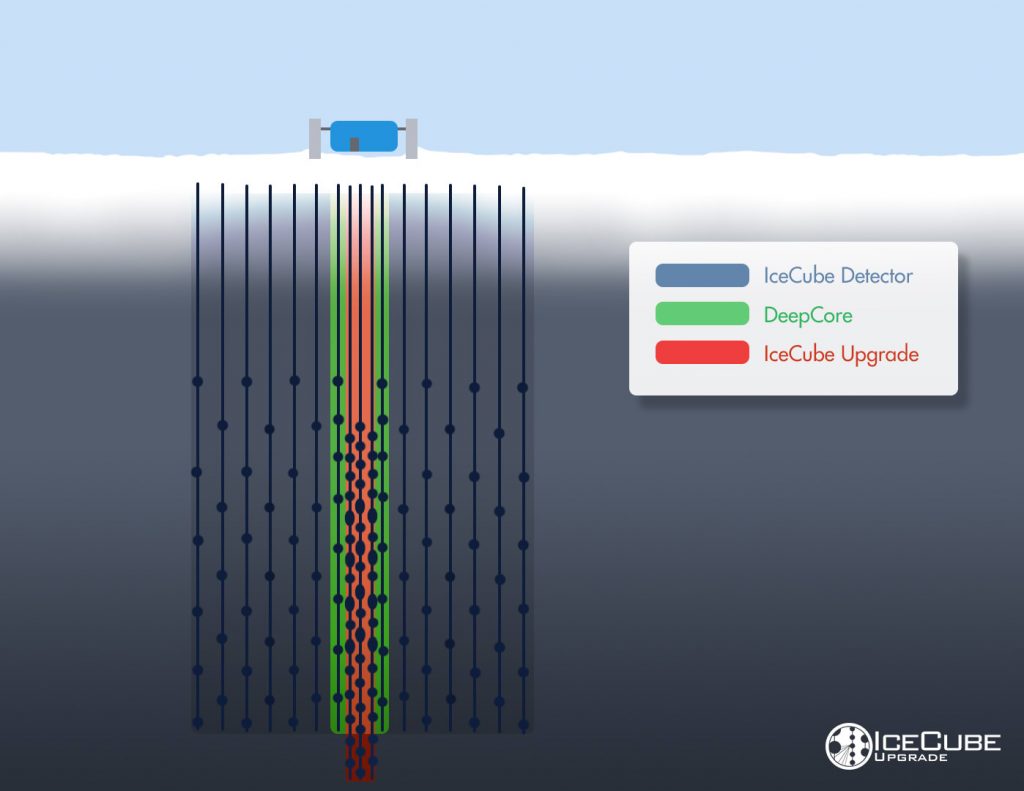
Bio
Carsten Rott is a Professor at the Department of Physics and Astronomy at the University of Utah, where he also serves as the Jack W. Keuffel Memorial Chair. He is also appointed at Sungkyunkwan University in South Korea.