John L Burba, PhD, Founder and CEO of International Battery Metals, details the fascinating 50 years of innovation he has been at the forefront of that has culminated in the company developing the world’s first commercial-scale modular and mobile lithium extraction plant.
Today, lithium is a very sought-after element due to its connection to high-energy batteries. In the last four years, we have seen a stunning surge in lithium demand that has completely overwhelmed the global lithium industry’s ability to supply. However, this is a very recent development.
In the 1950s, lithium was an exotic element. Its global production and consumption were very small, making it a true speciality chemical. During those years, lithium was used in glass compositions, thermally stable grease, organolithium compounds such as butyllithium, and thermonuclear weapons. Very few people believed that it would ever become a global critical metal or that it would be hugely important in fighting climate change. However, a small number of people saw the value in the metal, including Bill Bauman, who pursued lithium with a passion.
Dr Bauman was hired by the Dow Chemical Company during the Great Depression. He eventually headed Dow’s R&D laboratory in Midland, Michigan, and became the Vice President of Research and Development for the company. In the early 1970s, Bill stepped down from his Vice President position in Midland and became the first Dow Research Fellow. He set up a new laboratory in Texas Operations and began doing what he loved, research and development, specifically focusing on lithium.
I joined Bill’s lab in 1979 with a PhD in Physical Chemistry. Even then, he knew that lithium would be a dominant metal in the future. He reflected on a corporate meeting that he attended in the mid-1950s. At that time, the thought of people carrying telephones in their pockets was laughable due to the heavy lead-acid batteries used in them. Bill was instructed to try and remove this obstacle by Dow’s CEO, Leland Doan, subsequently producing a report on the best metal for a high-power battery based on electrochemistry and transport numbers.
He found that lithium was the optimal battery metal, and thus, he needed to find out whether there was lithium in any Dow-owned brine resources. Bill’s lab analysed brines in Michigan, Canada, and Arkansas, and lithium was found in all of these resources. Based on Dow Central Research Index Reports ‘CRI’, Dow began a systematic programme to recover lithium from these brines in the mid-1950s. This research continued until about 1968, when the programme was cancelled due to ‘insufficient progress.’
During this time, numerous efforts were conducted, including thermal evaporation to separate salts, chemical methods, and other processes. However, none of these efforts succeeded, and after the 1968 CRI report was presented to Bill, he terminated the programme. Dow had spent millions of dollars on its lithium programme, yet the company still did not know how to economically extract lithium from brine.
Work on the ‘lithium problem’
After Bill relocated to Texas, he began working on the ‘lithium problem’ with a vengeance. However, his approach to the problem was different. Rather than trying to use bulk chemical approaches, he focused on finesse. He and his team reasoned that if he could find something that would ‘grab’ lithium and only lithium, they could create a viable process to produce pure lithium products.
They took the approach of divide and conquer. John Lee, an organic chemist, focused on solvent extraction using a variety of ethereal solvents. Al Beale, PhD, conducted a massive literature search programme and theoretical chemistry. Ken Klaus, PhD, looked at materials that might capture lithium. Bill directed the work and focused on creating efficient separation systems.
Initially, John Lee’s work showed promise as he found a class of ethers that would strongly associate with lithium ions and transport them from the brine into an organic phase. The system worked very well with a simple sodium chloride/lithium chloride brine.
However, the technology ran into a brick wall when natural brines were tested. Calcium and magnesium ions were more strongly associated with the solvent than lithium. With real brine, the technology demonstrated very poor performance, and therefore, this approach was abandoned.
John and Bill tried every conceivable ion exchange system, including all of the known cation exchange resins, transition metal oxides, and zeolites, but none of these systems could provide the required selectivity for effective lithium recovery and impurity rejection. The issue was that the ion exchange selectivity coefficients for lithium, as opposed to all other cations in the brine, were simply too low.
The first real breakthrough came in the mid-1970s when Ken Klaus reviewed some of the lithium work conducted in the 1960s. In an attempt to recover lithium, a researcher added sodium aluminate solution to a sample of Michigan brine, and a gelatinous precipitate immediately formed. The report indicated that elevated levels of lithium, calcium, magnesium, sulphate, and chloride were found in the material.
However, they could not make a useable separation. Ken found that when he dissolved this substance with acid, the composition was predominantly aluminium, calcium, magnesium, and lithium. Further separation was not successful.
Ken then decided to ‘neutralise’ the material with acid, lowering the pH from around ten to about seven, rinsing the solids with hot water. The filtered solution was predominantly lithium, calcium, magnesium, chloride, and sulphate. However, their concentrations were low and all of the aluminium stayed in the solids. Bill then suggested that these solids be exposed to brine with agitation. After filtration and analysis, it was clear that some lithium had been transferred from the brine to the solids and could be recovered via washing with warm water.
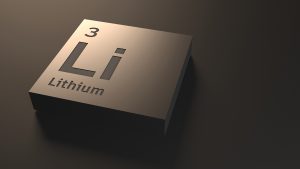
This was the first indication that direct lithium extraction (DLE) from brine might be possible. However, there were still significant problems as the solids were exceedingly fine. Each time they were mixed with brine, a portion of the solids dispersed in the brine solution and were unrecoverable.
After speculating on the problem, Bill and John decided to put the material into the pores of macro reticular ion exchange resin. First, they had to identify the appropriate macro reticular matrix, focusing on porous ion exchange resin. It was ultimately determined that Dow Macroporous Weak Anion Exchange Resin, MWA-1, was suitable. After numerous attempts, John found the appropriate method for loading the material into the resin. It was carefully neutralised and tested with lithium bearing brine. Lithium was absorbed and could be recovered with water. Furthermore, the composite resin could undergo numerous cycles without losing capacity. Several patent applications were filed to protect this invention.1,2,3
This successful step occurred just before I joined the group in May 1979. My background was in physical chemistry with an emphasis on surface science. John and I were given the assignment of improving the lithium resin production process. After about six months, we were able to synthesise composite resin that consistently extracted lithium from saturated brine.
There were two problems with the active material. While we knew the elemental makeup of the composite solids, and we knew that the composite resin effectively picked up lithium from the solution, we did not understand the structure of the inorganic material or the mechanism of lithium uptake. The only structural data that we had concerning the solids was X-ray diffraction data that was provided by Dow’s analytical lab. However, these solids were almost amorphous, so X-ray diffraction could not tell us much.
Walter Hensley, PhD, Crystallographer and Manager in the Dow Texas Operations Analytical lab, suggested that the material was likely a layered compound based on some very broad repetitive peaks. However, nothing else could be gleaned from the data.
Initially, we were convinced that the composite resin was a modified ion exchange system, with lithium associating with negative sites in an amorphous inorganic matrix. Anions were predominantly associated with the anion exchange resin. In essence, we thought that the amorphous material functioned as a cation exchanger in which lithium displaced sodium. In retrospect, it is easy to question our early mechanistic concepts.
However, in science, perspective is everything. In the early 1980s, our concepts were restricted by what we understood. In the spectrum of selective ion removal, our functional models were ion exchange systems and co-ordination compounds. These included ion exchange resins, chelating resins, zeolites, membranes, and solvents. We built our system models around these concepts.
Another issue that caused difficulty was that we were dealing with very concentrated and complex brine systems that contained only about 150 ppm of lithium. The total dissolved solids of the brine were 350,000 ppm, which obscured the issue.
Finding the correct path
I did not agree with the proposed mechanism for lithium uptake, so my primary focus was to find a better model. It was too difficult to work with small quantities of the active material held in resin pores, so I conducted experiments on pure material. Initially, I reproduced Ken’s work and then conducted numerous experiments to enhance the crystallinity of these solids. However, I was unable to grow crystals of adequate size from the amorphous gel.
After numerous attempts, I abandoned this approach and focused on highly crystalline analogues such as crystalline aluminium hydroxides. Samples were obtained from two commercially available aluminium hydroxide compounds, gibbsite and bayerite, and a nordstrandite sample was obtained from a geology professor in Europe.
These compounds are made up of stacks of thin sheets of aluminium hydroxide that maintain repetitive geometric patterns. Gibbsite, for instance, has a stacking pattern in which layers alternate in a repetitive ‘a-b’ pattern. Bayerite has a repetitive ‘a-b-c’ stacking pattern and the stacking pattern of nordstrandite is random.
My first experiment was to attempt to force lithium chloride into the spaces between the aluminium hydroxide layers. This was successful. The resulting compounds were very nicely crystalline. The stoichiometries of the compounds were very tight. Thus, we assumed the chemical formula, LiAl2 (OH)6 Cl.nH20, that applied to all three of the materials.
X-ray analysis provided very sharp patterns that demonstrated a well-defined stacking sequence. Furthermore, I could wash lithium chloride from the crystals with water and could also induce lithium chloride uptake from hot brine. At the time, I suggested using this material in a column for lithium extraction instead of the composite resin. This was rejected due to the very small crystal size of the compounds. Specifically, we experienced a very high-pressure drop in the laboratory columns when we operated with these compounds.4,5,6
The next question was about the structural locations of the lithium cation and the chloride anion. Since we could acquire excellent powder X-ray diffraction data of these compounds, we had a path to determine the location of anions in the system. However, lithium is the smallest metal ion in the periodic table. Due to its sparsity of electrons, it was essentially invisible to X-ray diffraction. Thus, we had to determine its location through inference.
I knew the stoichiometry of the compounds and understood an analogous group of aluminosilicate compounds that contained aluminium hydroxide layers, and effectively exchanged cations that resided between the sheets. These compounds are known as smectites, a class of ion-exchanging layered clay minerals. Cations will associate with sites on the clay surface where structural negative charges reside. Typically, these clays have sodium ions on the surface.
These ‘sandwich’ compounds have aluminium hydroxide octahedral layers sandwiched between silica tetrahedral layers. The aluminium hydroxide layers have aluminium ions in two-thirds of structural octahedra. This is very similar to gibbsite. It is also possible to force lithium ions into the other one-third of the holes through thermal treatment. However, this step in smectites is irreversible.
I determined that, when the lithium moved from the interplanar spaces into the octahedral sites in the aluminate layer of smectites, the material was inert and behaved like mica. It was impossible to disperse it in water or exchange ions on its surface.
I used this as an analogous system to the lithium aluminate compounds that I was making. I hypothesised that lithium occupied the unfilled octahedral sites in the lithium aluminate lattice. If this model were correct, the chloride ions would reside in the interplanar space between layers.
I tested this hypothesis by preparing lithium aluminate compounds with larger negative ions, such as sulphate, and then obtained X-ray diffraction patterns and measured the interplanar spacings. These spacings always matched the ionic radius of the corresponding anions, and thus, we concluded that the anions in these systems always reside in the spaces between the aluminium hydroxide layers.
This mechanism was highly unusual as it was not a charge-based system like ion exchange but a salt absorption system. Furthermore, it was extremely selective for lithium ions. We described the mechanism as ‘selective absorption.’
After the results of the experiments, Bill became very excited and focused the resin work on the formation of highly crystalline lithium aluminates. He also agreed that chloride ions in the system were associated with the crystalline lithium aluminate, not the ion exchange resin. This work resulted in significantly improved composite resin.
First Direct Lithium Extraction pilot plant
After a great deal of testing the improved composite resin with natural lithium-bearing brine, we designed a pilot lithium extraction plant that was placed at a Dow facility in Arkansas. The pilot lithium extraction plant was finished in 1983 and we began operations with natural brine that supplied Dow’s bromine operation. After dealing with typical start-up issues, we operated this equipment for about a year. Technically, the pilot work was successful. We were able to selectively extract lithium from the oilfield brine and we were able to demonstrate that commercial-grade lithium carbonate could be produced from this process.
These were positive outcomes, but the process did not meet financial goals and the project was ended. Dow was also in the process of divesting its bromine and calcium chloride operations. Thus, the first Direct Lithium Extraction plant effort ended, but the vision continued.
First commercial Direct Lithium Extraction plant
We were all disappointed when the Arkansas project ended. I stayed with Dow for another eight years and left for an opportunity at Great Lakes Chemical Company in 1992.
In 1993, Great Lakes was in dire straits due to the death of its CEO. Fortunately, I was recruited by FMC corporation to work in its corporate development group. An obvious attraction to me was that FMC corporation owned a lithium production business.
There were numerous interesting projects to work on and I dealt with interesting engineering problems, environmental problems, fires, and an explosion. However, the most germane work at FMC was the design of the world’s first commercial Direct Lithium Extraction plant.
FMC’s lithium extraction plant efforts began when they purchased Lithium Corporation of America (LITHCOA) in the early 1980s. LITHCOA’s spodumene mining operation was located at King’s Mountain, North Carolina, and the spodumene processing and product production plants were at Bessemer City, North Carolina.
In the late 1980s, FMC negotiated with the Argentine Government to acquire lithium extraction rights at Salar Hombre Muerto in the Andes with the intent of building a solar evaporation facility on that salar.
Solar evaporation is a process in which a series of lined, shallow, flat ponds are arranged in sequence. Fresh brine is pumped into the first pond, where halite, NaCl, crystallises and separates from the brine. In a clean brine, where there are low levels of borate and sulphate, subsequent ponds will precipitate potassium, magnesium, and calcium. Impure lithium chloride would be the final product.
Problems arise when the brine contains significant concentrations of anions such as sulphate and borate. These ions can enhance the solubility of polyvalent cations such as magnesium. When that occurs, the separation via evaporation is upended and the final product solution will contain much more magnesium and calcium than lithium. This leads to dreadfully low recovery rates and very high purification costs.
I was tasked with reviewing FMC’s solar evaporation design and was given samples of the salar’s brine concentration for analysis. The brine was predominantly a sodium, potassium brine with elevated concentrations of calcium and magnesium. However, it also contained sulphate and high concentrations of borate. Due to the common ion effect, I believed the magnesium would not crystalise.
These concerns were rejected and the pilot plant went ahead, yet the magnesium was not crystallising. To combat this, the team proposed the addition of lime to the solution to force the magnesium out as brucite, Mg(OH)2, which subsequently gelled the pond and rendered the pilot system impossible.
After this problem arose, Reg Hall, VP of FMC’s Specialty Chemical business, wanted to find a new solution, and asked about the viability of the technology that Bill and I had invented. I expressed that the technology had only produced small quantities of absorbent at that time. This time, however, we had to make it work.
We developed a plan with Reg that involved a significant effort in the FMC engineering lab. Engineers were brought from Argentina to the Princeton lab to work on the project, as they would run the plant when it was finished. We developed the critical processes, the process flow sheet, and an initial mass balance for the project.
FMC’s central engineering group began work on the design details and Fluor was selected as the contractor. The plant was finished and started up in 1998, consistently producing 99.5% pure lithium carbonate from the beginning.
In 1998, FMC’s plant at Salar Hombre Muerto in Argentina became the first commercial Direct Lithium Extraction plant in the world. Since that time, FMC has built several expansions, including the addition of a pre-evaporation pond that was designed to increase the lithium concentration in the brine. This plant stands as the world’s only commercial direct lithium production plant.
Path to modular and mobile
Since FMC’s plant start-up in 1998, I moved to other companies and technologies and the electrification revolution began. In 2012, I joined a start-up lithium production company named Symbol, which operated on geothermal brine in the Imperial Valley.
In 2014, we could not raise enough money to fund the construction and start-up of a plant and the company failed. The issue was that we needed $500m and about five years to construct and start a plant up. Furthermore, if we had experienced construction or operational issues, the timeline would have been longer. I began to recognise that the fatal flaw was that the cost and time to build and start plant operations were a serious mismatch with the capabilities of a young, poorly capitalised company.
After a lot of analysis, I concluded that the key to this problem was to design and build modular and mobile extraction plants. The key to accomplishing this is increasing process efficiency. Highly efficient small plants can produce as much product as larger inefficient plants. This became a seminal part of IBAT’s modular and mobile lithium extraction patent that was issued in January 2022.8
Key advantages of our modular and mobile design are:
Construction time
We can build a modular plant in fabrication shops that are located in industrial areas rather than in difficult and remote environments. For example, our first plant was built in two Fab shops in LaFayette and Lake Charles, Louisiana. We built our first commercial plant in ten months and assembled the plant in ten days. A conventional stick-built plant will require five to six years to construct.
Construction cost
Our construction cost is a fraction of conventional construction costs. This is particularly true for plants placed in remote, difficult locations such as Hombre Muerto.
Lithium production efficiency
There are two lithium production numbers that matter. Lithium extraction efficiency refers to the fraction of lithium that is removed from a particular lithium-bearing brine. The second number is lithium recovery efficiency. This number is actually a financially driven value. It refers to the fraction of the extracted lithium that can be recovered from the brine with adequate purity to be economically viable.
For example, a plant might be able to extract 95% of the lithium that is present in a given brine. However, due to poor impurity rejection, the useable lithium recovery efficiency may be only 40%. In this situation, the unrecoverable lithium goes out in the waste brine.
It is also important to realise that the lithium product recovery rate is a function of lithium concentration in the brine because the impurity profile is a function of the lithium-to-impurity ratio. The higher the lithium-to-impurity ratio in the brine, the higher the effective lithium recovery rate.
Environmental impact
IBAT’s technology represents a massive improvement in environmental impact. Existing lithium extraction plants all dispose of waste brine on the surface of their salars. This continually generates waste salt. It also depletes salar water tables. Furthermore, any process water that is added to the operation is also disposed of on the ground.
IBAT has developed an exceptionally advanced water recovery system that recycles about 98% of its process water. We do not dispose of water on the ground, and we do not draw massive amounts of freshwater from local aquifers. Furthermore, IBAT has publicly pledged to always operate in an ethical manner. This means that we will not waste water and we will minimise our carbon footprint at each of our operation sites. Additionally, we are particularly sensitive to the needs of Indigenous peoples that live around any brine resources that we produce lithium.
Construction and testing
We began construction of our first commercial-scale lithium extraction plant in June 2021. The plant was mechanically completed in December 2021. We assembled the plant at the Shaw Group Fabrication Facility in Lake Charles, Louisiana, in January 2022. This was done in ten days with nine people.
We began commissioning the plant in May 2022. After commissioning was complete, we negotiated an agreement with a company to provide natural lithium-bearing brine to our operation via tanker trucks. We began testing in June 2020. The system performed well, and we were able to selectively recover lithium from this brine.
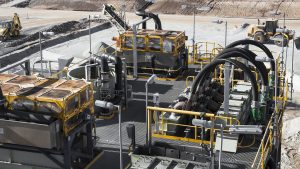
In July, we decided to test a brine from a different US source. The plant also performed well with that brine. In September, we engaged SLR, a well-regarded advisory and engineering firm, to independently evaluate and provide an opinion of our operations. The review and analysis were conducted by two SLR licensed professional engineers. They utilised the data that we developed over the summer and observed three days of operations. They did their own energy and mass balance analyses and provided a report that critiqued the design, construction quality, and operations of the plant.
SLR confirmed:
1. Robust design that lends itself to modularity and mobility;
2. Lithium extraction from the source lithium-bearing brine;
3. Recovery of quality lithium chloride from the absorbent, average recovery of lithium chloride product of approximately 68%. This rate is much higher than any commercial lithium from brine process in the world;
4. Efficiency of IBAT’s impurity rejection system;
5. Performance consistency through multiple cycles; and
6. Process water recovery based on real-time water balance calculations. SLR reported 94% recovery of process water through our primary recovery system. Unfortunately, our secondary recovery equipment had a failure and was unavailable. We project that, when this equipment is operational, we will recover an additional 4% of our process water.
International Battery Metals has constructed, assembled, and verified through independent engineering analysis the world’s first modular and mobile lithium extraction plant. This is a very important accomplishment.
Lithium product demand is expanding at such a rapid pace that our industry will not be able to positively impact the projected 50%-70% shortfall by 2030. There is simply not enough time to build conventional plants that may require 5-12 years to permit, build, and start-up.
Today, the only metric in this industry that really matters is time to market. IBAT has the solution to this problem.
References
- US 4116856 John M Lee and WC Bauman
- US 4348297A William C Bauman and John M Lee
- US 4348296A William C Bauman, John M Lee, John L Burba, III
- US4348295A, John L Burba, III
- US4727167A, John L Burba, III, William C Bauman
- US4464714A, John L Burba, III
- US5389349A, William C Bauman, John L Burba, III
- US11,229,880, John L Burba, III
Please note, this article will also appear in the twelfth edition of our quarterly publication.