A new research programme in low-energy nuclear structure, centred mostly on the High Intensity Gamma-Ray Source (HIγS), is currently under development at the Triangle Universities Nuclear Laboratory (TUNL).
A major goal of nuclear structure physics is to understand how nuclei are assembled, why some are stable and others decay, and how they interact with each other. The strong interaction that binds nuclei leads to a fascinating picture where the protons and neutrons manage to organise themselves and interesting phenomena emerge as a result, such as the appearance of nucleonic shells, manifestations of rotations and vibrations, and evidence for superfluidity and phase transitions. An essential contemporary challenge in this area is to understand how shell structure evolves on the proton- and neutron-rich sides of the valley of nuclear stability and to quantify the impact of this shell evolution on global nuclear properties such as the nuclear shape, the mass and binding energy, and the types of radioactivity.
Shell structure in nuclei
When it comes to understanding the structure of nuclei, physicists often state that ‘shell structure’ is the cornerstone of any satisfactory description. In analogy with atomic shell structure, shell closure results in enhanced stability. Thus, there are certain nuclei – those with a ‘magic’ number of protons and/or neutrons (2, 8, 20, 28, 50, and 82) – that have a full outer shell and are characterised by a large energy gap to the next available shell. As a result, they are more tightly bound (in terms of energy per nucleon) than nuclei with just one more or one less proton or neutron. For neutrons, there is an additional such number: 126. Nuclei that have either the proton or the neutron number equal to one such magic number are thus termed magic nuclei; doubly-magic ones are those with shell closures for both protons and neutrons. Recently, mostly from studies of neutron-rich nuclei, it has become clear that these numbers are not as immutable as once thought: in the exotic systems, some magic numbers disappear while others take their place.1
These observations highlight the fact that some component(s) of the nuclear force become(s) apparent at low excitation energy in nuclei with unusual proton-to-neutron ratios, away from the stable ones. However, this does not necessarily imply that such components are absent in stable nuclei. Rather, it is likely that these effects are weaker and that the impacted quantal states are located at higher excitation energy, in a domain poorly explored thus far. This is where the HIγS facility comes in. By scanning stable nuclei with photons of a precise energy, it is possible to identify with good precision nuclear levels all the way from the ground state to the point where energy is sufficient to eject a nucleon, and to determine the fundamental properties of these states such as their energy, quantum numbers, as well as their various deexcitation pathways (back to the ground state) and, possibly even lifetimes. With this approach, the research programme at HIγS, which focuses on stable nuclei, is complementary to research at other national and international accelerator facilities, where the emphasis is on exotic nuclei away from the stable ones.
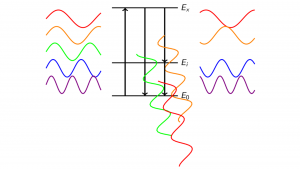
HIγS: the high intensity gamma-ray source
Nuclear structure research at TUNL relies mainly on high-flux gamma-ray beams from the HIγS facility. In its present configuration, HIγS is a laser Compton facility capable of delivering nearly mono-energetic gamma-ray beams with energies ranging from 1-100MeV, with both linear and circular polarisations greater than 95%. These polarised photon beams are a highly selective probe of the distribution of the electric charge and magnetism within the nucleus, and of the spin structure of nucleons. For example, for nuclear structure, linearly polarised γ ray beams enable the explicit determination of the spin and parity of excited nuclear states, and also allow the systematic study of correlations between the nucleons inside the nucleus. Other areas of research provide information relevant for nuclear astrophysics, low-energy tests of quantum chromodynamics (QCD), i.e., the description of the strong interaction that binds nuclei, etc. The photon beams are also used for numerous applications such as medicine and national security (for example Ref. 2).
The HIγS facility is currently the most intense laser Compton gamma-ray source in the world, reaching intensities in excess of 109 photons per second (Refs. 3 and 4). Operationally, HIγS is an electron-photon collider with two major components: an electron accelerator, that serves a dual purpose, and a free electron laser (FEL). In this system, high-energy gamma-ray beams are generated by Compton backscattering of photons (laser beam) from electron bunches circulating at relativistic energies inside a storage-ring based FEL. A layout of HIγS is shown schematically in Fig. 1. With a total storage ring circumference of about 108m, the FEL consists of four electromagnetic undulators that are situated in one of the two parallel sections of the racetrack-shaped storage ring. These undulators, along with a pair of high-reflectivity mirrors which are arranged to form to a 53.73m nearly concentric optical cavity, serve as the active elements of the FEL. To produce the laser beam, electrons, released from a photocathode microwave electron gun, are accelerated through a system consisting of a 180MeV pre-injector linac, a booster injector of 180MeV to 1.15GeV energy range, and a 1.2GeV storage ring. The oscillation of the electrons within the magnetic structure of the undulators results in the radiation of synchrotron photons in the forward direction of the electron beam. These photons, after amplification through a lasing process, collide head-on with a bunched beam of electrons to generate gamma rays via Compton backscattering. The energy of the resultant γ-ray beam, which has a spread of about 1% FWHM (by collimation), is tuned by adjusting the electron energy and magnetic field strength in the undulators.
A planned upgrade of the HIγS facility, HIγS2, is set to increase the γ-ray intensity by about two orders of magnitude at energies below 10 MeV, while providing beams with even better energy resolution.
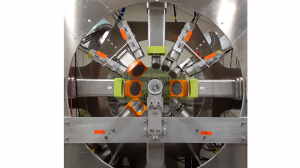
Nuclear resonance fluorescence and the clover array
The availability of polarised, quasi-monochromatic photon sources in the MeV range, such as HIγS, has led to a renaissance of nuclear resonance fluorescence (NRF)-based experiments.5,6 The process, illustrated schematically in Fig. 2, involves the absorption of a high-energy photon by a nucleus, herewith populating it to an excited state that will, subsequently, release the acquired energy in the form of one or several photons. Since the interaction with a photon is exclusively mediated by the well-known electromagnetic interaction, nuclear properties can be accessed directly without any assumption about the reaction mechanism.
Historically, this ‘clean’ method for nuclear structure studies employed photon beams generated as bremsstrahlung by a charged-particle beam. The transition from broad-band bremsstrahlung to quasi-monochromatic sources is comparable – if not as dramatic – to the revolution in atomic physics caused by the advent of the laser.
Present and future photon sources such as the VEGA system of the European ELI-NP,7 not only enable fundamental structure studies with unprecedented precision, but also make material assays possible in view of the fact that the NRF process is non-destructive.8
One challenge for NRF experiments is the low probability for photons to interact with matter at nuclear-resonance energies. In addition, the absorption of photons by narrow resonances competes with non-resonant scattering processes, such as Compton scattering, which can generate high backgrounds. This implies that, despite current photon sources providing beams of billions of photons per second, large isotopically-enriched samples with masses on the order of 1g have to be used and this currently limits the method to stable and sufficiently abundant isotopes.
The low interaction probabilities also impact the detection of the emitted photons following NRF. To take full advantage of the HIγS beam, the ‘Clover Array’ has recently been commissioned. This is the most sophisticated γ-ray detection system available at the facility for low-energy nuclear structure studies. It consists of up to 16 high-purity germanium (HPGe) detectors of the clover type (a single detector contains four separate germanium crystals in a clover-like arrangement), characterised by an excellent energy resolution (typically 2keV at 1MeV). In addition, up to 12 scintillation detectors of the relatively new, low-background cerium bromide (CeBr) material can be mounted. They complement the HPGe detectors by providing excellent timing resolution, well below one nanosecond.
Due to the high granularity of the setup, intricate quantum effects9 hidden in the rich electromagnetic radiation patterns of the gamma rays are accessible. Fig. 3 shows the array in its present configuration. Altogether, the system achieves a full-energy detection efficiency of a few percent, depending on the photon energy.
The power of the Clover array resides in part in its ability to require the coincident detection of multiple gamma rays. Not only does this capability enable the observer to delineate deexcitation paths involving cascades of several gamma rays between states in the nucleus, but it also helps to overcome the aforementioned background issue by discriminating in time the coincident cascades from the uncorrelated non-resonant reactions. Furthermore, if the nuclear states involved have lifetimes on the order of the timing resolution of the detectors, these lifetimes can be determined, herewith giving access to information often inaccessible by any other technique.
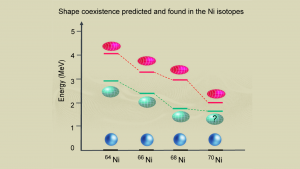
64Ni and the complementarity of measurements at HIγS and elsewhere
As an example of the complementarity between experiments at HIγS and those carried out at other facilities, recent results on the stable 64Ni nucleus are presented here.10 In the past few years, studies of the neutron-rich Ni isotopes with atomic mass units 66, 68 and 70 had discovered a phenomenon called ‘triple shape coexistence’, where three distinct shapes appear as energy is increased to the nuclei. In each case, the lowest-energy state is spherical, but the nucleus assumes flattened (oblate) and elongated (prolate) shapes as energy increases. Each shape reflects profound changes in the way protons and neutrons arrange themselves as a result of a component of the force (baptised the monopole tensor force) whose impact had not been fully realised until recently. It affects the energies of the nucleonic orbitals and weakens resistance to deformation.
The 66,68,70Ni investigations demonstrated that the deformed shapes come closer in energy to the respective ground states as more neutrons are added. Whether this component of the force is sufficiently strong to achieve shape coexistence in stable 64Ni was very much an open question when measurements at HIγS and at three other laboratories started. From the trend seen in the heavier Ni isotopes, the deformed states were expected to be higher in energy, making them harder to identify. However, the combination of mono-energetic beams at HIγS with the power of the Clover Array was instrumental in demonstrating shape coexistence.
As Fig. 4 indicates, roughly 3 and 4MeV are required to achieve oblate and prolate deformation, respectively, and the signal for the shapes should be expected to be weak at such energies. A tour de force took place involving four complementary experiments, jointly conducted by a collaboration between experimentalists from Romania, France, Italy, Poland, Romania, and the USA at four facilities, including HIγS. In addition to the NRF measurements, other reaction mechanisms were also employed including proton and neutron transfer, thermal-neutron capture, and Coulomb excitation.10 Taken all together, the data allowed to establish the existence of the coexisting ellipsoidal shapes, herewith providing important information required to better calibrate the monopole tensor force mentioned above.
References
- Otsuka, A. Gade, O. Sorlin, T. Suzuki, and Y. Utsuno, Rev. Mod. Phys. 92, 015002 (2020)
- R. Weller, M. W. Ahmed, and Y. K. Wu. ‘Nuclear Physics Research at the High Intensity Gamma-Ray Source (HIγS)’. Nucl. Phys. News 25,19 (2015)
- R. Weller, Prog. Part. Nucl. Phys. 62, 257 (2009)
- Yan, J. M. Mueller, M. W. Ahmed et al., ‘Precision control of gamma-ray polarization using a crossed helical undulator free-electron laser’. Nature Photonics 13, 629 (2019)
- Kneissl, H. H. Pitz, and A. Zilges, ‘Investigation of Nuclear Structure by Resonance Fluorescence Scattering’, Prog. Part. Nucl. Phys. 37, 349-433 (1996)
- Pietralla et al., Parity Measurements of Nuclear Levels Using a Free-Electron-Laser Generated γ-Ray Beam’. Phys. Rev. Lett. 88, 012502 (2001)
- https://www.eli-np.ro
- Lan et al., ‘Nuclear resonance fluorescence drug inspection’. Sci. Rep. 11, 1306 (2021)
- Beck et al., ‘∆K=0 M1 Excitation Strength of the Well-Deformed Nucleus 164Dy from K Mixing’. Phys. Rev. Lett. 125, 092501 (2020)
- Marginean et al., Phys. Rev. Lett. 125, 102502 (2020)
Professor A D Ayangeakaa
Dr U Friman-Gayer
Professor R V F Janssens
Department of Physics and Astronomy University of North Carolina at Chapel Hill Triangle Universities Nuclear Laboratory Duke University
+ 1 919 962-4703
+ 1 919 660-2636
+ 1 919 843-8168
ayangeak@unc.edu
ufg@email.unc.edu
rvfj@email.unc.edu
https://physics.unc.edu/people/janssens-robert