Dr Bhuvana Srinivasan, Associate Professor of Aerospace and Ocean Engineering at Virginia Tech (moving to the University of Washington in 2023), leads a team supporting several nuclear fusion energy concepts through computational modelling.
After approximately 70 years of scientific study, nuclear fusion is finally having its ‘Wright brother’s moment’, as recently described in the media. During this same period, there have been multiple revolutions in computing hardware, enabling predictive computer simulations and substantial scientific progress in a wide variety of scientific and engineering fields. Nuclear fusion is no exception to this.
The field of plasma physics, which is foundational for the success of nuclear fusion, has benefited immensely from the advances made in high-performance computing. Plasmas are considered the fourth state of matter, achieved when a gas is heated enough to break apart into its charged constituents, namely ions and electrons. The fusion fuel, which is ultimately expected to produce substantially more energy than the energy required to power a reactor, is plasma.
A comprehensive understanding of how this plasma behaves under burning fusion conditions and how to control this plasma is critical to the success of all fusion concepts. While multi-million or multi-billion-dollar experimental facilities may be limited in their ability to perform detailed iterative studies of plasma dynamics and stability, computational modelling can provide substantial insight towards design, prediction, and analyses and thus be a foundational pillar to the success of fusion energy. Computer simulations of plasmas require large supercomputers and highly efficient and accurate models to capture detailed physics at highly disparate spatial and temporal scales, spanning many orders of magnitude, for example, from hundreds of metres down to micrometres in a magnetic fusion device.
These computational advances have enabled higher and higher fidelity models to offer insights that were not possible in the previous century and, thus, have provided paths to fusion that were not previously evident. As public and private entities pursue different paths to nuclear fusion, one could not overemphasise the importance of computational modelling to the success of all fusion concepts.
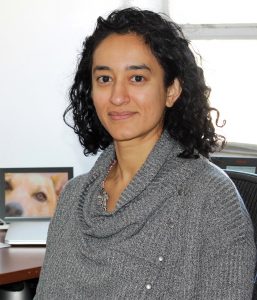
Dr Bhuvana Srinivasan leads a team of plasma physics students, postdoctoral researchers, and research scientists performing computational modelling in support of multiple fusion energy concepts that include inertial confinement fusion, magneto-inertial fusion, magnetic mirror concepts, plasma-jet merging concepts, Z-pinches, and tokamaks. Three of her team’s efforts are highlighted here.
Plasma-wall interactions for any device where the plasma interacts with a solid wall
One of the major challenges in fusion energy is to develop materials that can withstand the temperatures, particle fluxes, and heat fluxes that are encountered when they are in contact with hot plasmas. For context, fusion reactors are expected to produce plasma temperatures above 100 million degrees Celsius, about ten times hotter than the centre of the Sun. Nearly all materials become vaporised at temperatures above 5000 degrees Celsius.
As a first step, one needs to quantify what temperatures and fluxes are encountered at these material walls and whether there is a way to cleverly manipulate plasma-wall interactions to mitigate the impact on the plasma and the wall. Together with collaborators at Princeton Plasma Physics Laboratory and Los Alamos National Laboratory, Dr Srinivasan’s team is working towards understanding the microscopic behaviour of the plasma very close to a material wall. This situation is encountered in any device that bounds a plasma with a solid wall. These not only include a wide variety of fusion devices but also spacecraft propulsion devices that use plasma as a propellant. A plasma sheath forms in a localised region near the wall because electrons, the negatively charged constituents in a plasma, are much lighter and faster than even the lightest ion, which is the positively charged constituent of the plasma. Since electrons outrun the ions, an electrostatic potential (similar to a voltage) develops self-consistently to slow down the electrons and accelerate the ions. Although the physics inside of a sheath is microscopic, it can have macroscopic effects on the global plasma even far away from the wall, and importantly, it can provide information on how the wall material may be impacted. This can aid in the development of novel wall materials and methods to control the fusion fuel to ameliorate detrimental plasma-wall interactions.
Dr Srinivasan’s team is working on fundamental sheath studies that apply to multiple fusion configurations including tokamaks, mirror machines, and z-pinches, in research supported by the U.S. Department of Energy’s SciDAC (Scientific Discovery through Advanced Computing) and ARPA-E (Advanced Research Projects Agency-Energy) BETHE (Breakthroughs Enabling Thermonuclear Fusion) programs. Incorporating higher fidelity physics can notably impact predictions of plasma transport or the exchange of mass, momentum, and energy, and including such transport models can fundamentally impact sheath behaviour. This has led to significant contributions to fundamental sheath theory highlighted in recent publications.1,2 One of the preliminary findings from Dr Srinivasan’s team is that, as the currents and voltages in z-pinch reactors are increased towards fusion powerplant regimes, the anode, which supplies current to the device, may become especially susceptible to damage from high particle and heat fluxes.3
Ionisation and recombination (breaking isotopes of hydrogen into a plasma and vice versa) and other interactions that result when ions and electrons collide with each other, between themselves, and with the neutral hydrogen isotopes can also significantly modify the near-wall behaviour of fusion devices. Accurate modelling of these mechanisms may be leveraged to study regimes where fusion devices perform more stably and with reduced damage to the wall. Dr Srinivasan’s team continues to develop higher-fidelity models that incorporate detailed collision models into plasma simulations. Additionally, because the plasma is inevitably at significantly higher temperatures compared to those that most materials can withstand, wall material is emitted to the plasma in the form of electrons, ions, and sputtered particles. The impact of emitted wall material into the fusion plasma can reduce the temperatures that are necessary to achieve a burning fusion plasma, making studies of the impact of the wall emission on the plasma a critical aspect of Dr Srinivasan’s research.4,5 Her team is studying how plasma dynamics are affected by emission and whether novel wall materials can be employed to protect both the walls and the fusion plasma.
Plasma-wall interactions for any device where the plasma interacts with a liquid wall
Together with her co-Principal Investigators at Virginia Tech, Dr Colin Adams and Dr Stefano Brizzolara, Dr Srinivasan and her team are exploring how a liquid wall may mitigate the material limitations of using a solid wall as a plasma-facing component. This research is being supported by the ARPA-E BETHE program. Several fusion concepts plan to eventually replace their solid first wall that comes into contact with the plasma with a flowing layer of liquid that may be able to withstand far higher particle and heat fluxes without concerns of material damage. The liquid wall is sometimes envisioned to be a liquid metal that could also conduct electricity and thus serve as an electrode to supply electric current to the plasma.
The Liquid Electrode Experiment (LEX) at Virginia Tech is being led by co-Principal Investigator Dr Adams as a part of the ARPA-E BETHE award and is presently providing validation data for computer simulations of the liquid metal response to fusion-relevant impulses. Some fusion configurations require high currents ranging from many hundreds of kilo-Amperes to mega-Amperes being driven from electrodes to the plasma. If one were to replace solid electrodes with liquid electrodes, the high currents may result in significant dynamic motion of the liquid that may include ejecta, droplets, waves, and wave-breaking. In a scenario such as in a nuclear fusion power plant, the current pulse must be repeated up to ten times every second for energy production, and the liquid needs to settle down to a quiescent state between each pulse. The Virginia Tech team has discovered that, depending on the strength of the current pulse that is applied, the liquid metal can react very violently, raising several questions about how the liquid wall will impact the performance of a fusion reactor. A study of this behaviour and potential mitigation strategies is one of the core competencies of this ARPA-E BETHE team. The experimental and computational efforts can be generalised and modified to suit many different fusion experiments with different liquid wall configurations.
Despite the ability to study plasma-liquid wall dynamics experimentally, computer simulations are necessary for this area. Liquid wall materials that are excellent candidates for fusion reactors, such as liquid lithium, react strongly with water and produce highly combustible hydrogen. Experimental laboratories must use special precautions when researching such materials. Experiments using other liquid metals which present minimal hazards can provide physics insights that can be applied to more hazardous wall materials. Secondly, experiments are yet to access fusion reactor conditions; however, insights from experiments at the lower energies can be extended to much higher energy scenarios in computer simulations, enabling the study of regimes that a liquid wall may encounter in a powerplant-scale nuclear fusion reactor. This way, computer simulations can access regimes for predictive capabilities that are not presently accessible through experiments. This ARPA-E BETHE team is working on a number of upcoming publications highlighting their experimental and computational findings from this work.
High-energy-density plasma fusion concepts towards the development of inertial fusion energy
The recent success at the National Ignition Facility in achieving fusion ignition has been remarkable for the fusion community as it has highlighted to the world that the path to controlled nuclear fusion, and eventually to a fusion reactor powerplant, is indeed attainable. Inertial fusion energy requires a rapid compression of fusion fuel, often through the use of lasers or other pulsed-power drivers. These approaches fall within the category of high-energy-density inertial confinement fusion concepts, where pressures exceed one million atmospheres during controlled implosions. One of the major challenges in these concepts is to overcome the undesirable mixing of hot and cold fuel that can reduce the temperatures necessary to achieve fusion breakeven and the higher fusion yields necessary for nuclear energy production.
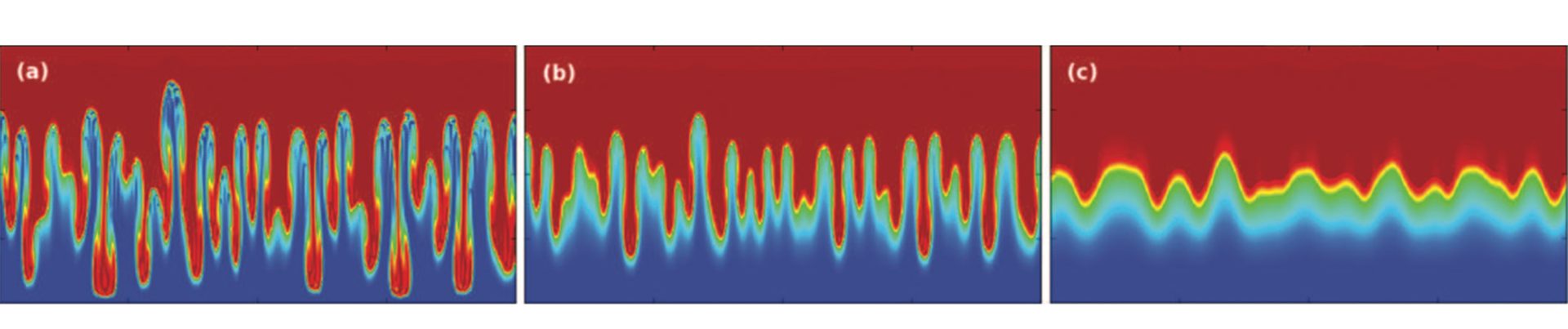
Dr Srinivasan and her team have performed fundamental studies to inform how this mixing occurs, the importance of detailed physics models to understand such mixing, and what options may exist to mitigate this mixing to achieve higher fusion yields. Consider the simple example provided in Fig. 1.6 Plot (a) presents an example of undesirable mixing where a heavy fluid is positioned above a light fluid and falls due to acceleration. This process is analogous to what would occur if a jar containing water and oil were suddenly inverted or accelerated downward. Plot (b) shows that the inclusion of viscosity in our computer simulations can modify the predictions of the mixing behaviour. Plot (c) shows how including an appropriately oriented magnetic field of sufficient magnitude can substantially reduce the mixing. This work has been extended in several follow-up publications and continues to be studied by Dr Srinivasan and her students experimentally at the Omega laser facility at the University of Rochester and also through computer simulations.7,8,9,10 These studies suggest avenues towards higher yield inertial fusion energy through the use of applied magnetic fields to reduce mixing.
Dr Srinivasan’s team performs computational modelling of plasma physics relevant to many different fusion concepts and her team continues to contribute to the community’s efforts to achieve powerplant-scale nuclear fusion reactors through high-fidelity computer simulations.
References
- Yuzhi Li, Bhuvana Srinivasan, Yanzeng Zhang, and Xian-Zhu Tang. “Bohm criterion of plasma sheaths away from asymptotic limits.” Physical Review Letters 128, no. 8 (2022): 085002
- Yuzhi Li, Bhuvana Srinivasan, Yanzeng Zhang, and Xian-Zhu Tang. “Transport physics dependence of Bohm speed in presheath–sheath transition.” Physics of Plasmas 29, no. 11 (2022): 113509
- R. Skolar, K. Bradshaw, J. Juno, and B. Srinivasan, “Continuum kinetic investigation of the impact of bias potentials in the current saturation regime on sheath formation”, Physics of Plasmas 30, 012504 (2023)
- Petr Cagas, Ammar Hakim, and Bhuvana Srinivasan. “Plasma-material boundary conditions for discontinuous Galerkin continuum-kinetic simulations, with a focus on secondary electron emission.” Journal of Computational Physics “406 (2020): 109215
- Kolter Bradshaw, Petr Cagas, Ammar Hakim, and Bhuvana Srinivasan. “Plasma sheath studies using a physical treatment of electron emission from a dielectric wall.” arXiv preprint arXiv:2210.14117 (2022)
- Bhuvana Srinivasan, and Xian-Zhu Tang. “Mitigating hydrodynamic mix at the gas-ice interface with a combination of magnetic, ablative, and viscous stabilization.” Europhysics Letters 107, no. 6 (2014): 65001
- Bhuvana Srinivasan, Guy Dimonte, and Xian-Zhu Tang. “Magnetic field generation in Rayleigh-Taylor unstable inertial confinement fusion plasmas.” Physical review letters 108, no. 16 (2012): 165002
- Bhuvana Srinivasan, and Xian-Zhu Tang. “The mitigating effect of magnetic fields on Rayleigh-Taylor unstable inertial confinement fusion plasmas.” Physics of plasmas 20, no. 5 (2013): 056307
- Ratan Kumar Bera, Yang Song, and Bhuvana Srinivasan. “The effect of viscosity and resistivity on Rayleigh–Taylor instability induced mixing in magnetized high-energy-density plasmas.” Journal of Plasma Physics 88, no. 2 (2022): 905880209
- Samulski, B. Srinivasan, MJ-E. Manuel, R. Masti, J. P. Sauppe, and J. Kline. “Deceleration-stage Rayleigh–Taylor growth in a background magnetic field studied in cylindrical and Cartesian geometries.” Matter and Radiation at Extremes 7, no. 2 (2022): 026902
Please note, this article will also appear in the thirteenth edition of our quarterly publication.