Dr Anita Cochran, Assistant Director of the McDonald Observatory at the University of Texas at Austin, is exploring the evolution of our Solar System by studying comets
Our Solar System formed 4.567 billion years ago, when a cloud of gas collapsed to start the formation of the Sun. As the gas compacted, any rotation of the gas would cause a flattened disk to form around the ever-more dense central condensation. When the density of the gas was sufficiently hot (around one million degrees), the fusion of hydrogen into helium would start, therefore powering the star, our Sun. At this point, the force of gravity causing the central star to collapse is offset by the outward pressure of the gas from heating, causing a stable Sun. The disk of material around the Sun would then start to have mass concentrations that would gravitationally attract more material forming clumps, initially centimetres and subsequently kilometres in size. These clumps are called ‘planetesimals’. The temperature of the disk is not constant, the closer to the star, the higher the temperature. Thus, the planetesimals show a range of compositions based on the temperatures at which they formed. Planetesimals near the Sun would be predominantly made of rock; planetesimals far out in the Solar System would be icy. These planetesimals would clump together more via slow collisions, building up bigger bodies – the planets.
Of course, we could not watch all of this happen, so how did we formulate such a scenario? The picture has been built up over time by observations of comparable systems and by studying the components of our Solar System. There are regions of our galaxy that are just beginning to form stars. We can observe clouds of gas of different ages and see various stages of the processes. From such studies, we can conclude that typical protostellar disks only last between five and ten million years (a blink of the eye to an astronomer or geologist). Thus, we know that the window of time in which planets can form is only five to ten million years. After that, the disk is depleted (mostly blown away by the outflow of gas from the star, although some of the disk has been converted into planetesimals), and the growth of the star and planet discontinues. The bigger planets will gravitationally affect the orbits of about 90% of the remaining planetesimals by a process called gravitational perturbations and will also sweep some up to encourage growth. By doing so, they ultimately escape the gravitational pull of the Sun and are lost from our Solar System.
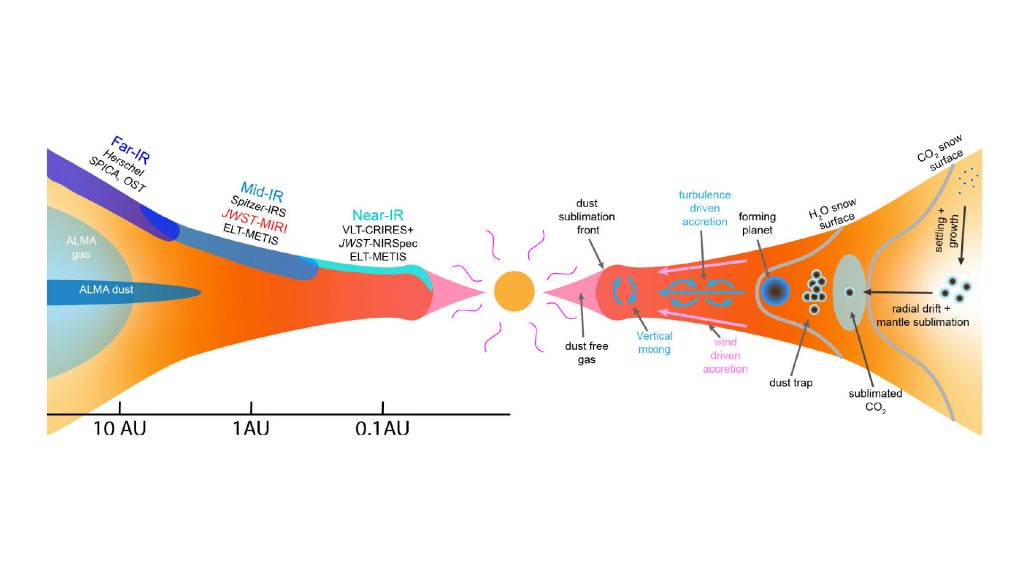
Protostellar systems
When we observe protostellar systems, we can only obtain limited measurements of the conditions in the disks because they are far away, meaning that we cannot obtain much spatial information. From these observations, however, we do get an idea of how the temperature of the disk varies in relation to its distance from the star and we can also determine that the disk is thicker in the outer regions. But we cannot pinpoint detailed information on temperatures at all points in the disk, nor can we ascertain the chemical composition of the whole disk because the disk is optically thick in places, i.e. we cannot see the inner material because our view is blocked by the outer material.
To put together more information, we must revert to observations of objects in our Solar System. However, the planets are of limited use for this because they have evolved substantially during the history of the Solar System. For example, when the Earth was formed, there was a very limited atmosphere and no life. We had an active body, however, that was molten in parts due to residual heat from its formation as well as interior radiogenic heating. The resulting convective motion of the Earth’s mantle drives the plate tectonics that cause earthquakes and tsunamis.
Eventually, a secondary atmosphere was formed. Lifeforms started to emerge about 3.5 to 4.0 billion years ago; macroscopic multi-cellular life began about 1.5 billion years ago and first transitioned to land about 500 million years ago. Conditions such as volcanoes, storms, plate tectonics, and life have all influenced the appearance of the Earth as we see it today. Thus, if we want to understand the conditions at the origin of our Solar System, we have to be able to model out all of those processes, including some we might not be aware of. What is true of Earth is also true of all the planets in our Solar System.
In order to try to unravel these complications, we need to study objects that formed at the start of our Solar System but which have changed very little. For an object to undergo minimal changes, it must not be too large, meaning that it has no active geological processes such as tectonics or weather; it must be in an orbit that keeps it relatively far from the Sun; and it must not have life. Such objects do exist. They are the 10% of the remnant planetesimals that did not escape from the Solar System. These include asteroids in the inner Solar System and comets in the outer Solar System. As the comets stay much colder than the asteroids due to their distance from the Sun, they are the least altered objects following the formation of the Solar System and are thus the objects that can lend pertinent constraints to our understanding of that formation.
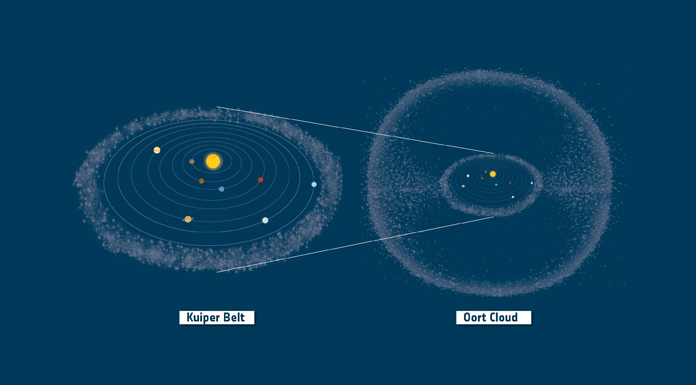
Illustration showing the two main reservoirs of comets in the Solar System: the Kuiper Belt, at a distance of 30-50 AU from the Sun, and the Oort Cloud, which may extend up to 50,000–100,000 AU from the Sun
Comets
Today, comets reside in one of two reservoirs. The first of these is called the Oort Cloud, named after Jan Oort, an astronomer who, in 1950, discovered that a spherical halo of comets (the leftover icy planetesimals renamed) must surround our Sun. This halo stretches to about 100,000 AU, where we define 1 AU, or astronomical unit, as the mean distance of the Earth from the Sun.
The Sun’s gravitational field is becoming too weak to hold comets in orbit due to the distance between them . These comets did not form in the Oort Cloud. Instead, they formed in the region of the giant planets (Jupiter to Neptune) and make up the 10% of planetesimals that did not get completely ejected from our Solar System by gravitational perturbations. The second of these reservoirs is called the Kuiper Belt which exists from the orbit of Neptune to around 1000 AU. Gerard Kuiper realised that planetesimal formation would not magically stop at the orbit of Neptune and that there must be leftover planetesimals past Neptune that never formed a planet. Unlike the Oort Cloud, which is a spherical halo surrounding our Solar System, the Kuiper Belt is a disk of bodies. In both cases, the comets are in orbit around the Sun, the same as the planets. Those in the Kuiper Belt orbit in the same direction as the planets. However, the gravitational perturbations that cause comets to move from the giant planet region to the Oort Cloud scramble the orbits so that there is no preferred orientation relative to the planets. Thus, comet Halley orbits the Sun in the reverse direction from the Earth’s orbit around the Sun (termed retrograde), while comet Hale-Bopp’s orbit is perpendicular to the orbit of the Earth around the Sun.
Comet injection
A comet is basically a dirty snowball. They are small bodies (generally one to 25km in diameter) that are composed of a 50:50 mixture of ices and dirt. About 80% of the ices are water ice, with the remainder composed of methane, ammonia, carbon monoxide, carbon dioxide, methanol, ethane, acetylene, formaldehyde, etc. The dirt (generally termed ‘dust’ in comets) is essentially the same as rocks, gravel, sand, and silt on Earth. As long as the comets stay in one of their reservoirs, the ices stay in their solid form and the comets remain mostly unchanged. However, occasionally, a comet is dislodged from its reservoir and its orbit then takes it into the inner Solar System, where we can observe its behaviour.
One way to dislodge a comet and send it to the inner Solar System is from a star passing through the Oort Cloud. However, models of stellar motions within our galaxy have shown that only about 5,500 stars have passed within 100,000 AU of the Sun in the lifetime of the Solar System. These passages occur with a typical velocity of ~20km/sec and can eject any comet within about 450 AU of the passing star. Thus, passing stars have probably ejected (both towards and away from the Sun) about 10% of the Oort Cloud comets.
The larger influence on Oort Cloud comets that will send them into the inner Solar System is the tidal field of our galaxy acting on the disk. As our Sun orbits the centre of our galaxy (about every 250 million years), the pull of the mass of objects near the centre of our galaxy perturbs the Oort Cloud. This causes the Oort Cloud to actually be a prolate spheroid (rather than a sphere), with axes of around 100,000 AU for objects orbiting in the same direction as the planets (prograde) and around 120,000 AU for retrograde orbits.
When the comets enter the inner Solar System, they are heated due to being closer to the Sun. In the near vacuum of space, the ice does not get converted to liquid but instead is transformed directly to its gas state through a process known as sublimation. The small nucleus of the comet has little gravity so cannot hold onto the gas, meaning that it escapes. The escaping gas also carries with it some of the dust. This escaping gas and dust form an envelope around the nucleus called the coma, which then obscures our view of the nucleus. It is this coma, and any resulting tails that form, that create the beautiful objects we study as comets in the inner Solar System.
Observations of comets in the inner Solar System have been recorded for as long as the written history of civilisations on Earth. It was Sir Edmond Halley in the 1700s who realised that some comets visit the inner Solar System repeatedly. However, it was not until the 1850s that the first studies of the composition of comets were undertaken, with early spectra. Indeed, in those first spectra, there were many species that were detected that could not be identified because the laboratory studies were not sufficiently robust to interpret the comet spectra. Even without knowing exactly what all of the species in the spectra were, it was obvious that most cometary spectra looked the same, with only subtle differences. Did this mean that all comets formed under the same conditions and/or in the same location? Were conditions very uniform over a wide range of Solar System distances?
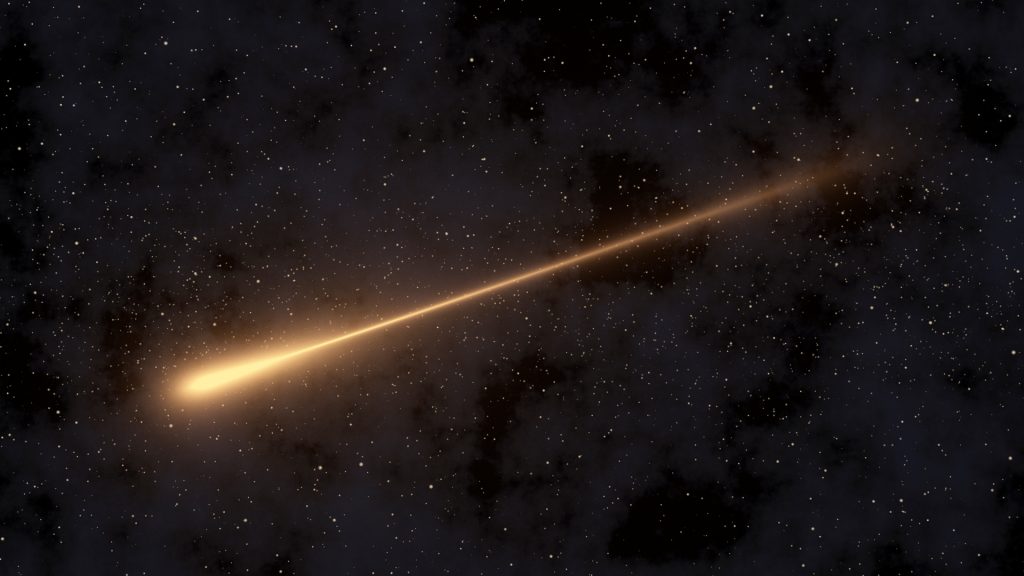
It is impossible to tie individual comets to the place that they formed because we cannot trace them from their current orbit uniquely back to their formation location
Developing a better understanding of comets
Coupled with a better understanding of the dynamics and reservoirs for comets, several groups set out to determine whether all comets were indeed chemically similar or whether there were differences that we could detect. These studies started in the 1970s in the optical region of the spectrum and have since been extended into the IR, UV, and radio wavelengths as technology has improved.
In the optical, it was soon apparent that the spectra of most of the comets were indeed very similar, with the major difference being how much dust was in the coma. Various ices volatilised at distances that made sense for the temperature of that region of the Solar System. But these ‘typical’ comets were not the only type of comets that we observed – they only represented about 75% of the objects we studied. The other 25% seemed to be missing some species or, more correctly, had only very small amounts of them. These species were ones with long strings of carbon, such as acetylene or propyne (inferred from the dissociation products we see once the sunlight has dissociated the gases that originate from the nucleus ices). These comets are now called ‘depleted’ comets. However, it was not all carbon-bearing species that were missing; hydrogen cyanide relative to water was the same in these ‘depleted’ comets as in the ‘typical’ ones.
As the statistics increased and other wavelength regimes were included, the first thought was that we had two reservoirs for comets, the Oort Cloud and Kuiper Belt, and two types of chemistry. Were the ‘depleted’ comets from one reservoir and the ‘typical’ from the other? Unfortunately, the picture is not that simple, as we find ‘depleted’ comets from both reservoirs, with a higher percentage of Kuiper Belt comets being ‘depleted’ than Oort cloud comets and both reservoirs having ample ‘typical’ comets, too.
A complication in turning such studies of the compositions of comets into an understanding of the chemistry of the early Solar System is that we are not directly observing the nucleus of the comets. We observe the gas phase of the material that has sublimed. Once in the gas phase, it can undergo chemistry in the densest regions of the coma nearest the nucleus and can be ionised and dissociated by sunlight as it flows outwards. Indeed, in the optical part of the spectrum, we are observing only (daughter) products of the chemistry and photo processes and not any of the ices that occupy the nucleus; we must infer the original ices from models. In the IR and radio, we do see some primary (parent) species, but identifying which species are the original ices can be complicated. In order to disentangle this puzzle, we must compare optical observations with IR, radio, and UV observations, drawing from teams all around the world with access to different types of telescopes.
The statistics of many objects observed at many wavelengths paint a picture of the conditions that existed at the distances at which the comets formed. However, it is impossible to tie individual comets to the place that they formed because we cannot trace them from their current orbit uniquely back to their formation location. We must rely on dynamical models to infer the likely formation zone we are probing.
Even when inter-comparing observations at different wavelengths, our observations are only sensitive to certain features and we must model our observations to infer the true nucleus composition. Thus, we have sought ‘ground truth’ by flying robotic space missions to comets to study them in more detail. The first missions to comet Halley in 1986 (from Europe, Japan, and the Soviet Union) enabled us to measure the size, get preliminary chemistry of the gas and dust, and showed us that the nucleus was blacker than would be expected from pure ices. The latter was due to the admixture of dust with the ice (think about how bright white snow becomes duller as dirt is added). A subsequent mission (the Stardust mission) to comet Wild 2 by NASA brought back dust samples that we measured in the lab to show that cometary dust was indeed very primitive. A NASA spacecraft (Deep Impact) collided with comet Tempel 1 to show that the nucleus was very porous (about 80% porosity), resulting in a bulk density that is lower than that of water ice, and was homogeneous to substantial depths.
The Rosetta mission
The most substantial mission to date has been the ESA Rosetta space mission to comet Churyumov-Gerasimenko (CG). The spacecraft rendezvoused with CG around 3.5 AU and stayed with the comet as its orbit took it to its closest approach to the Sun. CG is far from a spherical body; it has been likened to the shape of a rubber duck! Along the way, the spacecraft used a variety of cameras, spectrographs, thermal detectors, and mass spectrometers to study how the comet evolved with time. In addition, the main spacecraft released a small auxiliary spacecraft, Philae, that landed on the surface (after first bouncing a few times) to measure the properties of the surface. With the Philae observations we confirmed that the nucleus was very porous and therefore did not conduct heat into the interior of the nucleus well. This confirms that little of the interior should have changed from when the comet was formed.
From orbit, the composition was measured in great detail with the mass spectrometers and dust analysers. These observations confirmed that CG is a ‘depleted’ comet and amplified that classification with many details. This ties back to the statistical studies of many comets to pull together a more global picture of cometary composition that is grounded on the detailed observations of this one comet. What is still unknown is how representative CG is of comets as a whole and where exactly it formed. More missions would be desirable, but they are generally expensive. ESA and NASA are both seriously considering missions to rendezvous with a comet and bring back a sample of the nucleus. This enables a direct measure of nucleus material. However, unless the sample is brought back cold (called a cryogenic sample return), chemistry within the retrieval vessel still could take place and would need to be modelled.
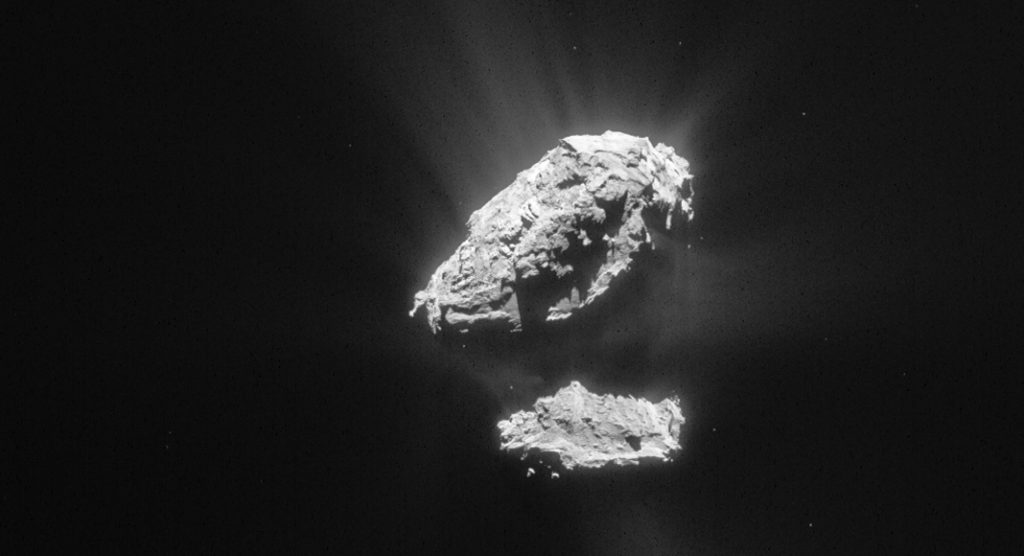
Comet 67P/Churyumov-Gerasimenko in May 2015, the same month that Rosetta also made the first detection of the organohalogen methyl chloride
An incomplete picture
Putting together decades of ground-based data, spacecraft data, and dynamical models, we have created a picture of what materials would have existed at different regions of our Solar System at the time the Sun and planets formed. It is a picture with holes that still need exploring by astronomers with access to facilities around the world. However, sometimes this exploration can lead to unexpected pieces of evidence that need to be factored into our picture.
One such surprise came in 2017 when Dr Adam McKay and I discovered that one comet, C/2016 R2 (PanSTARRS), was not like either of our two categories of comets. Instead of being 80% water ice, it was 80% CO ice and had almost no water. It also had a very high ratio of N2 to CO. Where did this object form and why is it different? Most of the comet observing community jumped to get observations of this target at all different wavelengths. The CO abundance and lack of water were confirmed, and other weird details discovered. Theorists have now been challenged by this comet. And the community is now happily contemplating if this object is unique (probably not) and what it implies for our picture of how our Solar System formed.
Dr Anita Cochran
Assistant Director
McDonald Observatory
The University of Texas at Austin
anita@astro.as.utexas.edu
Tweet @UTAstronomy
www.as.utexas.edu/astronomy/people/cochran
Please note, this article will also appear in the sixth edition of our quarterly publication.