Dr Vincent Tisserand, Research Director at Clermont Ferrand Physics Laboratory (LPC PLUS), looks at the past, present, and future of efforts to make a precise measurement of the CP violation phase to challenge the Standard Model of Particle Physics.
I prepared my PhD thesis in the CERN ATLAS experiment, at the LAL Orsay laboratory near Paris, where I was a part of the group that designed the electromagnetic liquid argon accordion calorimeter detector. That detector is a key ingredient in what was used to discover the Higgs boson into final states, including high transverse energy photons and electrons. Right after, in 1998, I was enrolled at CNRS in the French LAPP Annecy laboratory, located near CERN. Since then, I have been conducting research in the field of elementary particles, more precisely on what physicists call ‘heavy flavours’ (i.e., hadronic particles composed of ‘heavy’ quarks, while the so-called ‘light’ quarks are the elementary components of ordinary matter such as protons and neutrons).
My main contributions are related to the study of the decays of beautiful mesons (featuring a heavy quark called beauty, discovered in 1977 by the team of Professor L Lederman at Fermilab in Chicago). I have participated in the global effort by the worldwide community of physicists to better compel and test the description of the material-antimatter asymmetry of the Standard Model (SM) of particle physics in the quark sector. This approach is essential to understanding the discrepancy between the prediction of the SM and the matter-antimatter asymmetry observed in cosmology, which is of several orders of magnitude higher.
The composition of matter
Just as several musical notes are required to complete a musical composition, the same is true for the composition of matter, with known matter being made of 12 elementary fermion particles: six leptons and six quarks. Meanwhile, the forces are carried by 12 bosons, four for electroweak interactions (photons, W±, and Z0) and eight for strong interaction (gluons), and in addition a special one, the Higgs boson, which is responsible for the mass of those elementary particles.
The cosmological model of the Big Bang, or the expansion of the Universe, was introduced by physicists A Friedman and G Lemaître in the 1920s, following Einstein’s theory of general relativity. It was later experimentally confirmed by astronomer E Hubble in 1929, and again in 1965 by A A Penzias and R W Wilson. The fact that our Universe is mainly made of matter is then a quite long-standing enigma confronting the Big Bang theory, which naively predicts that the same amount of matter and anti-matter was evident in the early Universe. The fact that matter and antimatter obey different physical laws (violation of the discrete CP symmetry, where C and P stand for charge parity conjugation) is necessary for the formation of the Universe’s constituents, from nuclei to celestial bodies (stars and planets, i.e., after ‘baryogenesis’) and, therefore, essential for our existence. This condition was formalised by the Russian theorist A Sakharov in 1967, who described it in a short and illuminating reference paper.
Matter-antimatter asymmetry
Therefore, for more than a century, physicists have been developing the concept of matter-antimatter asymmetry within the SM of elementary particle physics. This fundamental science is paved by many theoretical and experimental works that have been awarded numerous Nobel Prizes in physics.1 This includes the fascinating discovery of the anti-electron, the ‘positron’, in 1932 by C D Anderson (i.e., the first ever observed antiparticle), preceded by the equations of P A Dirac. This was followed by the major milestone observation of the violation of the symmetry of left-right parity in weak interaction (P symmetry violation), in 1956, by S C Wu. The beautiful Wu experiment, dealing with cold 60Co atoms, is a way to operationally define left and right without reference to the human body. Such a non-expected behaviour had been previously predicted by T D Lee and C N Yang.
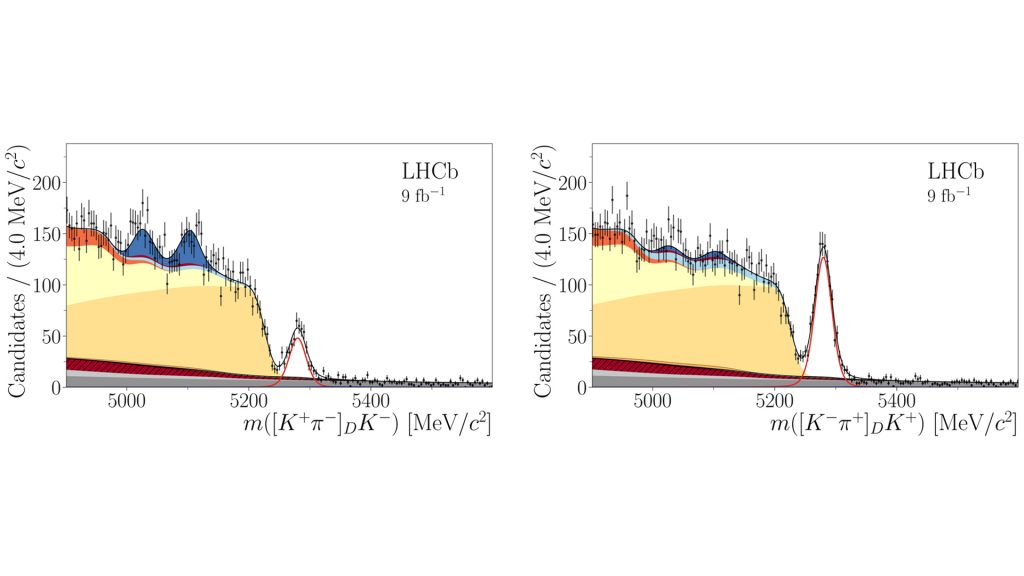
The actual direct discovery of this matter-antimatter asymmetry (CP violation) dates back to 1964 and the work of J W Cronin and V Fitch and their team on neutral-K mesons, ‘kaons’, made of strange quarks. They observed 45 anomalous events from a total of 22,700 detected decay events, a ratio of about one in 500. Already from that result it became clear that CP violation is a relatively rare phenomenon. Since the beginning of the Big Bang, there has been a fight between matter and antimatter, where doublets of particle and antiparticles annihilate into gamma photon pairs and vice versa. While decaying, matter particles slowly (less than three minutes!) but surely dominated against antimatter in the early Universe.
Following a pioneering study by the Italian physicist N Cabibbo, published in 1963, and the 1970 discovery of the Glashow-Iliopoulos-Maiani mechanism, in 1973, the two Japanese theorists M Kobayashi and T Maskawa proposed an extraordinary prophetic scenario to explain the CP violation phenomenon seen in kaons. This relies on the existence of three other yet unknown quarks:
- The charmed quark (discovered during what physicists call ‘the revolution of November 1974’); and
- The beauty and top quarks, discovered respectively in 1977 and in 1994 at Fermilab, near Chicago.
Such a scenario was presented as a minimal field content scenario based on simple arguments of complex algebra and group theory. They thus introduce into the SM a phase that generates an interference between quarks and anti-quarks, which is responsible for their abundance symmetry breaking, the effects of which are irreversible.
Their description of quark decays and interactions implies a mixing complex unitary matrix, named after the initials of the above three physicists with the acronym ‘CKM’. Such a matrix is a 3×3 matrix that sizes the magnitude of the coupling of up-type quarks (u, c, t) and down-type-quarks (d, s, b) and it can be parametrised by four fundamental constants: λ (the Cabibbo angle), A, and a complex number (ρ+iη), which is known as the CKM angle γ. One usually illustrates the constraints on the complex number (ρ+iη) with a triangle called the CKM unitarity triangle.
It was not until 2001 that the discovery of CP violation seen in 1964 was repeated with neutrals-K mesons with neutral-B mesons with the BaBar experiment at Stanford, to which I contributed, and its competitor Belle at Tsukuba in Japan. Subsequently, and thanks to BaBar and Belle collaborations (‘B-factories’), Kobayashi and Maskawa were awarded the Nobel Prize in Physics in 2008, which was described as being ‘for the discovery of the origin of the broken symmetry which predicts the existence of at least three families of quarks in nature.’
In 2019, CERN’s LHCb made this observation again with neutral-charmed mesons. The ATLAS and CMS experiments at the LHC have also been looking for it with the top quarks. Such discoveries have been obtained with hadrons made of quark pairs, and a wonderful breakthrough would be an observation of CP violation with more complex objects made of three quarks: the baryons, as protons and neutrons, which constitute the nuclei of atoms, which are building blocks of molecules and which of course, are built upon themselves ever upwards until we see the macroscopic constituents of the Universe.
An accurate measurement of a non-zero value of the CKM angle γ is therefore partial proof of the validity of the KM mechanism. While it is a fundamental constant of Nature, it is certainly more interesting for a physicist to perform an accurate measurement of γ than be simply motivated by metrology. Below I will explain why that is, but first, let’s discuss how that is achieved.
The non-zero value of γ
It took BaBar and Belle almost a decade and a little over a billion of b/anti-b pairs produced (by the PEP-II and KEK-B accelerators), to measure γ as being equal to (67±11)°,3 combined, with some 15 observables measured with charged-B mesons decaying into the final states D(*)K(*)±. The non-zero value of γ was thus firmly established a decade ago at more than five standard deviations.
The sensitivity to γ in B± to D K± decays is strong, and methods exist to enhance the CP asymmetry of detected final states D K- with respect to D K+ (see GLW, ADS, GGSZ, GLS after the initials of their theoretical authors).2 For those modes, counting an asymmetry of the number of detected B- (anti-matter made an anti-u and a b quark) versus that of B+ (matter made of a u and an anti-b quark) is an evidence of direct CP violation. The phenomenology of those decay modes is relatively simple as they occur at what we call ‘tree level’. They certainly represent the most SM-like hadronic decays in heavy flavours.
Experimentally they are quite challenging because, for such methods, one must deal with decay rates of the order of 1ppm or less and detection efficiencies of the order of a few per cent. Efficient detectors based on performant triggering, vertexing, tracking, and particle identification systems are required.
At the LHC, the LHCb experiment has been operating since 2011 and took data until 2018. A few hundred billion b anti-pairs have been produced by the LHC at the LHCb interaction points. Fig. 1 displays a relative spectacular CP asymmetry of about 45% of matter versus antimatter for one of the above modes and methods! Together with about 100 other observables, at the 2020 edition of the yearly workshop ‘Implications of LHCb measurements and future prospects’ the LHCb presented a global combination that determines the CKM angle γ to be equal to (67±4)°.5 LHCb is from far driving the world average. There are more existing data and methods still not yet published. As opposed to B-factories, LHC does not only produce B mesons; it also produces all the kinds of b-hadrons: Bs (pair of b and s quarks) and Bc (pair of b and c quarks), b-baryons (a b and two other quarks). Measurements with Bs mesons have not yet been performed or are underway, and CP violation was not yet observed in beauty or charm-baryons.
One may ask why it is still important to improve the precision further. The answer is because the direct measurement of the CP violation phase γ from B± to D K± decays are theoretically clean and constitute a reference for the global coherence test of the KM mechanism within the SM. While including any observables that constrain the parameters, but those above described for the measurement of γ, λ (the Cabibbo angle), A, and a complex number (ρ+iη), the CKMfitter group, for which I am one of the experts for γ, determines from a global fit an indirect value for the CKM angle γ that is equal to (65.7+0.9−2.6)°.6 Such an indirect determination is based on observables potentially sensitive to virtual quantum mechanics effects due to new heavy particles of physics beyond the SM. Those non-standard effects infer through the Heisenberg inequalities, where energy conservation can be violated during a sufficiently short time interval (ΔE.Δt ~hbar). It is known that the SM is an effective theory at the few hundred GeV scale. As the direct determination is yet much less accurate as its indirect determination, about 4° against about 1°, it is highly desirable to pursue the quest of precision. CKMfitter demonstrated, with a sub-degree precision level, that one may probe the validity of the SM to energy scale at the level of a few tens of TeV, that is beyond the capability of direct searches by ATLAS and CMS experiments.
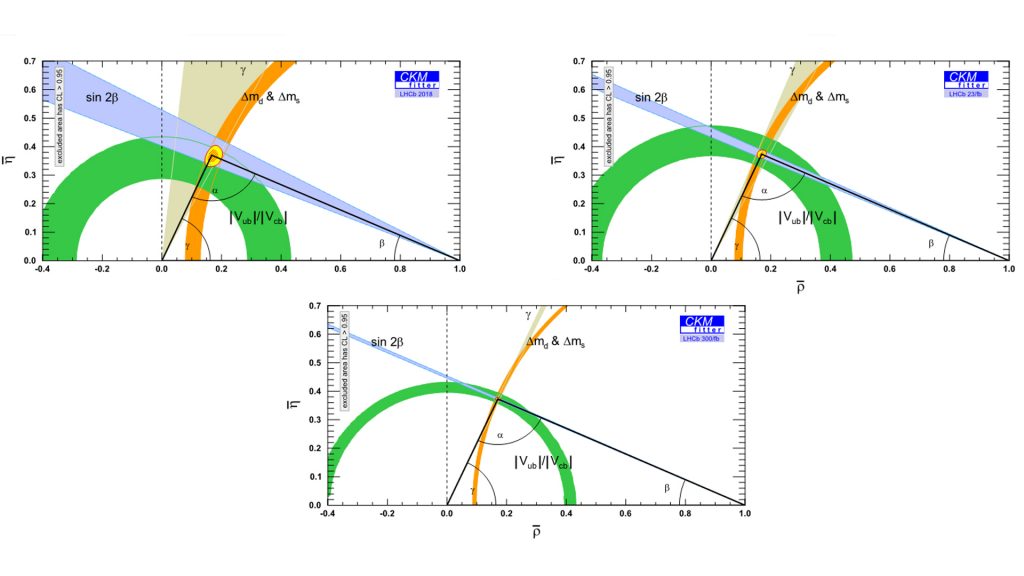
The upgraded LHCb
Since 2011, the LHCb experiment has undergone R&D activities for a major upgrade effort of its detector.7 The new detector is being assembled and commissioned and should be able to take data at the LHC restart in early 2022. The main improvement of the LHCb detector is based on a new data acquisition where the low-level triggering is designed to cope with a rate of 30MHz, while until now it was operated at 1MHz. The vertexing, tracking, and particle identification systems are also brand new. Among the various new sub-detectors, the LPC Clermont-Ferrand LHCb group has been participating to the new scintillating fibres (SciFi) tracker project, especially for the frontend and readout boards and their mechanical housing. Fig. 2 shows an illustration of the assembly of the SciFi readout board in August 2020 at CERN.
To improve the statistics of the rare hadronic B decays, such as B± to DK±, which again is a key parameter to improve the precision on the direct γ CKM angle, the upgraded LHCb detector will be operated, after 2022, with an instantaneous LHC machine luminosity at the interaction point of 2×1033 cm-2.s-1, so five times more than that of the 2011-2018 period. The LHCb collaboration is also looking forward to a second upgrade to be deployed in ten years from now, in order to operate at 1.5×1034 cm-2.s-1. The present available dataset corresponds to an integrated luminosity of nine fb-1, it should raise up to 50 and 300 fb-1, after upgrade phases one and two, respectively. Therefore, one may anticipate a precision on the direct measurement of γ of the order of 1-1.5°, by 2025-2026, and 0.3-0.4°, by 2035-2038. The expected ultimate precision provided by Belle II, which began operating a few months ago in in Japan, is 1.5°. I computed, with the CKMfitter software, the expected impact of the LHCb experiment on the summit (ρ+iη) of the CKM unitarity triangle since now and after the upgrade phases 1 and 2. This is displayed in Fig. 3 and extracted from reference seven.
References
- The Experimental Foundations of Particle Physics, R. N. Cahn and G. Goldhaber, Cambridge University Press (2009)
- Ceccucci, T. Gershon, M. Kenzie, Z. Ligeti, Y. Sakai, and K. Trabelsi, [arXiv:2006.12404 [physics.hist-ph]]
- J. Bevan et al. [BaBar and Belle collaborations], ‘The Physics of the B Factories’. Eur. Phys. J. C74 (2014) 3026
- Aaij et al. [LHCb Collaboration]. LHCb-PAPER-2020-036, CERN-EP-2020-225, arXiv:2012.09903 [hep-ex] submitted to JHEP
- W. Kenzie and M. P. Withehead [on behalf of the LHCb Collaboration], LHCb-CONF-2020-003, CERN-LHCb-CONF-2020-003
- The CKMfitter Group (J. Charles et al.). Eur. Phys. J. C 41, 1 (2005) [arXiv:hep-ph/0406184], updated at [ckmfitter.in2p3.fr], Phys. Rev. D 89, 033016 (2014) [arXiv:1309.2293 [hep-ph]]
- [LHCb Collaboration], CERN-LHCC-2012-007; LHCb-TDR-12 and CERN-LHCC-2018-027, LHCB-PUB-2018-009 [arXiv:1808.08865 [hep-ex]]
About the author
Since 1994, Dr Vincent Tisserand has contributed to more than 1,000 publications (around 500 for the LHCb collaboration at CERN (Geneva), around 500 for the BaBar collaboration at SLAC (Stanford), six at ATLAS, and five at CKMfitter).
Following a thesis at the Linear Accelerator Laboratory in Orsay on the ATLAS experiment of the Large Hadron Collider (LHC, CERN, Geneva), Tisserand joined the CNRS at the Laboratory of Annecy-le-Vieux of Particle Physics in 1998, where he was the LHCb group PI from 2012 to 2017. He then chose to join the LHCb group at the Clermont Physics Laboratory in 2017. He first conducted his research in the BaBar experiment at Stanford and then, from 2010, in the LHCb experiment. He has been a member of the international phenomenological group CKMfitter since 2005.
Both experiments are devoted to the study of heavy flavours, albeit under quite different conditions, with BaBar being located on an electron-positron collider, and LHCb being operated at the proton-proton LHC collider.
He has contributed to studies of heavy flavours at various stages: working on instrumentation; the development of analytical tools, especially for neutral particles; complex, varied, and complementary analyses of beautiful mesons decay channels in both experiments. He was also part of the BaBar core team of physicists that observed the violation of the CP symmetry with B mesons.
For over 15 years he has been working on the precise measurement of the phase as predicted by M Kobayashi and T Maskawa in 1973.
Laureate of the 2019 Joliot-Curie Prize of the French Society of Physics
Please note, this article will also appear in the fifth edition of our quarterly publication.