SLAC National Accelerator Laboratory is leading groundbreaking advancements in fusion research through innovative technologies, aiming to tackle the challenges of harnessing fusion as a viable energy source.
Ever since the discovery that our Sun produces energy by fusing light atoms together to release heat, the prospect of reproducing that same process here on Earth to power our homes and industry has captured the minds of scientists.
Following decades of fusion research dating back to the 1950s, it was only in December 2022 that a breakthrough occurred: a laser experiment on the National Ignition Facility (NIF) became the first laboratory fusion event to release more energy that was used to initiate it. This milestone, along with other promising approaches, has driven significant private investment in fusion energy, but many challenges still remain before fusion can be scaled to a viable power source.
The High Energy Density Science (HEDS) division at SLAC National Accelerator Laboratory is at the forefront of tackling these obstacles. Leveraging SLAC’s unique capabilities, the HEDS division collaborates with academic and National laboratory partners to advance inertial fusion energy (IFE) as a potential global energy solution.
The innovative contributions of SLAC’s LCLS and MEC
SLAC National Accelerator Laboratory has a long history of pioneering scientific advancements. Established in 1962 to house the Stanford Linear Accelerator, then the largest civil construction project ever sponsored by the US, SLAC’s work has contributed to three Nobel Prizes in particle physics.
Over time, as higher-energy accelerators such as CERN’s Large Hadron Collider took the lead in particle collision experiments, SLAC pivoted to new applications. One of its most transformative initiatives came in the early 2000s when a portion of the accelerator was repurposed into the Linac Coherent Light Source (LCLS), the world’s first X-ray Free Electron Laser.
LCLS revolutionised X-ray science by producing beams billions of times brighter than any other X-ray source. These ultrafast, high-intensity pulses enabled researchers to capture ultrafast processes in materials in real-time. Scientists worldwide have since used LCLS to resolve questions of the Natural sciences, such as photosynthesis, the development of next-generation painkillers, and the structural determination of viral proteins. The success of LCLS has inspired the construction of similar X-ray Free Electron Lasers in multiple countries, marking a new era in scientific research.
Together with the high-power optical lasers available at the Matter in Extreme Conditions (MEC) instrument, LCLS has also launched a new era of precision measurement of plasma and fusion science, allowing researchers to probe ultrafast changes in matter under extreme conditions.
At MEC, optical lasers create pressures comparable to the centre of the Earth and temperatures higher than the solar corona. Meanwhile, the X-ray beam penetrates these extreme states, providing a window into their dynamic evolution to test our ability to predict the conditions in the interior of planets, stars, and laboratory fusion plasmas.
Advancing X-ray diagnostics for fusion science
A critical aspect of fusion research is the ability to directly probe matter as it reaches high-pressure, high-temperature conditions. X-ray diagnostics provide essential insights into these states:
- X-ray diffraction: By capturing atomic-scale structural changes, X-ray diffraction lets us see how atoms rearrange in materials under extreme compression. For example, experiments with laser-driven plastic samples have revealed how hydrocarbon chains split apart and reassemble into diamonds at conditions similar to those within Uranus and Neptune. These findings help refine models of planetary formation and could also motivate new technical applications on Earth, such as using laser-formed nanodiamonds for biomedical and industrial purposes.
- X-ray scattering: This technique provides a more detailed view of interactions within high-temperature states. By measuring how X-rays gain or lose energy upon scattering, researchers can directly determine sample temperatures – offering insights that previously relied on indirect modelling. Recent studies using this method have deepened our understanding of how our planet’s magnetic field is generated and shed light on how materials respond to pressure and radiation and how they can be improved for use in fusion and space explorations.
- X-ray imaging: The nanometer spatial-scale resolution of LCLS enables ultrafast imaging of laser-driven samples. Researchers from our division have observed the lattice dynamics of compressed silicon and how instabilities form as electrons flow through hot plasma. More recent advances have allowed simultaneous imaging and diffraction, providing a more complete picture of material transformations.
The future of fusion science at SLAC
With the advent of LCLS-II, SLAC is poised to push the boundaries of fusion research even further. This major upgrade includes a superconducting accelerator capable of delivering millions of X-ray pulses per second. It introduces a unique pulse train feature, enabling sequential X-ray snapshots with variable time separations to capture rapid sample evolution in a single shot. The upgrade will also double the maximum X-ray energy, penetrating into samples with heavier atoms and at higher densities. These advancements will allow researchers to track the behaviour of fusion fuel capsules in unprecedented detail, moving closer to understanding the conditions necessary for sustained fusion reactions.
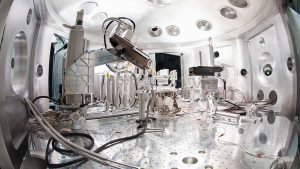
One of the key challenges in high-repetition-rate fusion experiments is delivering targets at a pace that matches the laser shot rate. While earlier experiments at LCLS used static arrays of a few hundred targets, new approaches involved continuous streams of liquid jets, suspended nanoparticles, or cryogenic hydrogen, enabling experiments at far higher rates and giving us enormous datasets to learn from. These innovations support the development of next-generation fusion targets and plasma diagnostics, ultimately enhancing the efficiency and viability of laser-driven radiation sources.
All of our diagnostics will play a part in exploring the physics of fusion capsules. By the end of the decade, we are planning on an upgraded laser system at the Matter in Extreme Conditions endstation, capable of driving samples to the brink of fusion. As samples are driven by kJ-scale laser pulses, we can track the sample from its initial solid state to the high-energy-density physics regime that characterises the inertial fusion plasma state. Our diagnostics will need to work together to understand optimisation pathways, with X-ray diffraction probing the fuel density, scattering looking for temperature growth, and imaging looking for instabilities and inhomogeneities.
Sequential X-ray pulses will apply these capabilities to study how matter traverses into and through the high-energy density phase space, even when the initial target has unique characteristics such as defects, inclusions, or texture. Machine learning can combine data from these diagnostics to narrow down the conditions that are reached on the timescale of the shot, uncovering the underlying physics mechanisms that determine the coupling of high-intensity laser beams with matter.
While LCLS offers unique opportunities for X-ray probing, our division uses beams from across the electromagnetic spectrum to understand fusion targets. Optical probes can use interferometry to see how quickly a laser-driven shockwave moves through fusion fuels, while the intensity of emission tells about the temperature that the compressed target reaches. Terahertz radiation, with wavelengths close to 1 mm, allows measurements of electrical conductivity, influencing how electric fields are generated and propagated inside the extreme states, which is essential for fusion approaches that use magnetic fields to drive their compression. As with the range of X-ray diagnostics, our experiments have to pull together the data from all of these different measurements to understand the heating and laser coupling to fusion targets. Beyond that, generation of relativistic electrons, high energy X- and γ-rays, magnetic fields, and energetic particle beams are all areas where probing with the LCLS X-ray beam will lead to new discoveries in areas of critical importance to fusion energy science development.
Collaborating for a fusion-powered future
Beyond diagnostics and experimental advancements, SLAC’s HEDS division collaborates closely with theorists and computational scientists to refine models of fusion dynamics. By comparing real-world experimental data with simulations, researchers can identify gaps in theoretical understanding and drive improvements in predictive models. Even as the NIF laser can reach the most exciting conditions, high-resolution data from LCLS complements that and serves as a crucial benchmark.
Looking ahead, the challenges of designing a functional fusion power plant extend beyond the reaction itself. The fuel – a mix of deuterium and tritium – must be reliably contained and delivered at high repetition rates. Unlike NIF, where fusion fuel is encased in perfect diamond shells, meticulously grown over months, a commercial fusion plant would require target production at a rate of ten per second.
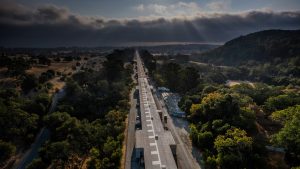
To meet this need, SLAC, in collaboration with the U.S. Department of Energy’s Inertial Fusion Energy Network (IFE-STAR) and the Fusion Innovation Research Engine Collaboratives (FIRE), is developing and characterising novel lightweight foams capable of holding cryogenic fusion fuel. Early LCLS experiments have already examined the structural integrity of these foams, with future studies planned to analyse how they hold the cryogenically cooled liquid fuel and how the wetted foams behave under laser compression.
Finally, the success of fusion power will depend on materials that can withstand the intense neutron bombardment produced by the deuterium-tritium fusion reactions. SLAC researchers are actively working with materials scientists to develop radiation-resistant alloys, using LCLS to assess how their properties change under extreme conditions, and as their atoms are transmuted by the high-energy neutrons. These efforts will inform the design of reactor walls capable of withstanding prolonged exposure to fusion-driven neutron fluxes.
Conclusion
As SLAC’s fusion research progresses, each step brings us closer to unlocking the power of the stars for clean, sustainable energy. With cutting-edge X-ray diagnostics, high-energy-density physics experiments, and deep collaborations across scientific disciplines, the HEDS division is paving the way for a future where fusion energy becomes a practical reality. By refining experimental techniques, enhancing theoretical models, and addressing engineering challenges, SLAC is contributing to one of the most transformative scientific endeavours of the 21st century.
Please note, this article will also appear in the 21st edition of our quarterly publication.