International Editor Clifford Holt spoke to Imperial College London’s Dr Jongseok Lim who, having recently been awarded a prestigious Ernest Rutherford Fellowship, plans to measure the shape of the electron by using an array of molecules cooled to microkelvin temperatures, potentially enhancing our knowledge beyond the standard model of particle physics.
It is widely accepted that the Standard Model of particle physics is incomplete because it fails to explain several important observations. To build a more complete picture of the universe, physicists are striving to reveal what lies beyond the Standard Model. Much of the research being done at particle accelerators such as the LHC at CERN is working towards this objective. But measuring the shape of an electron can be used as an alternative way to explore the same problem.
Lim, who has recently been awarded an Ernest Rutherford Fellowships by the Science and Technology Facilities Council (STFC), will therefore use this opportunity to build an apparatus that uses an array of molecules cooled to microkelvin temperatures to make an extremely precise measurement of the electron’s shape.
International Editor Clifford Holt spoke to Lim about how, with very careful measurements, such table-top experiments will enable him to probe energies equal to, or even above, those reached by the particle accelerators.
Could you begin by explaining how measuring the electron could help to solve the baryon asymmetry problem? What progress has been made towards answering the question of the matter/antimatter imbalance at large particle accelerators such as that at CERN?
The Standard Model of particle physics is one of the greatest achievements of modern science. It has been fabulously successful in classifying the fundamental particles and explaining how they behave with amazing accuracy. Nevertheless, it is widely accepted that the Standard Model is incomplete because it fails to explain several important observations. One prominent example is the excess of matter over antimatter in the Universe. The Standard Model predicts almost equal amounts of matter and antimatter, but observations show the Universe contains only matter. This contradiction is one of the great unsolved problems in modern physics and a major deficiency of our most fundamental theory.
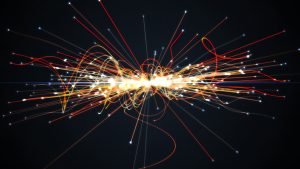
To build a more complete picture of the Universe, physicists are striving to reveal what lies beyond the Standard Model. It is known that the matter/antimatter asymmetry requires violation of the Charge Parity (CP) symmetry. There is CP-violation in the Standard Model, but it is far too small to explain the observed matter/antimatter asymmetry in the Universe.
Many extensions to the Standard Model have been proposed that address such deficiencies, and these theories are tested in current high-energy physics experiments. At CERN, researchers are looking for new particles with high energy that could potentially cause matter/antimatter asymmetry. The first creation of atoms of antimatter has opened the door to the systematic study of antimatter, and anti-hydrogen was first captured in 2010 and then used to compare physical properties of atom and anti-atom with amazing accuracy.
LHCb experiments aim to discover clues to the unsolved mystery more directly. This is where most of the particle physics CP-violation studies are happening at the moment. Recently, the LHCb collaboration observed time-dependent matter/antimatter asymmetry. They also measured tiny mass difference between particles when D0 meson changes from matter to antimatter and back again.
There is an alternative, and ingenious, way to explore the same problem – measure the shape of an electron. One of the generic features of the new theories that extend the Standard Model is that they assume there exists new, undiscovered particles carrying CP violation to explain the matter/antimatter asymmetry. The new source of CP-violation makes electrons slightly non-spherical. This distortion – known as the electric dipole moment (eEDM) – changes the energy of an electron in an electric field, and that tiny change is amplified when the electron is bound to a molecule.
My project aims to build an apparatus that uses an array of molecules cooled to microkelvin temperatures to make an extremely precise measurement of the electron’s shape. With very careful measurements, such table-top experiments enable us to probe energies equal to, or even above, those reached by the particle accelerators. Such marvellous precision has become possible by my recent success in cooling molecules to ultracold temperature. That technique is the foundation of my project.
How will cooling an array of molecules to microkelvin temperatures allow for an extremely precise measurement of the electron’s shape? What techniques etc. will you employ? What will this achieve that large particle accelerators like the LHC cannot?
The strong potential to discover new physics makes eEDM experiments a vibrant research frontier. In all previous eEDM experiments, the eEDM is detected through the precession of the electron spin in an applied electric field, which is greatly amplified through relativistic effects when the electron is bound to a heavy polar molecule such as YbF.
Generally, the statistical sensitivity in such experiments is inversely proportional to the spin-precession time. In current eEDM measurements using a molecular beam, the spin precession time is limited to about one-thousandth of a second. Over the last decade, there have been a handful of eEDM experiments using heavy polar molecules, improving the eEDM sensitivity by three orders of magnitude. The improvement over the past decade is incredibly impressive, but the measurement precision of these experiments has reached the achievable limit using the previous design.
Therefore, innovative methodologies are required to take the EDM search into the future. My plan is to dramatically increase the spin precession time, which is the key to improving EDM sensitivity, by four orders of magnitude by using ultracold molecules trapped in an array. This is an ambitious challenge, but it is achievable by using the latest advances in ultracold molecular physics.
To decelerate a beam of YbF molecules to rest, I will use the forces exerted by carefully tuned laser light. Then, I will trap these molecules in a magneto-optical trap and cool them to a few microkelvin before loading them into an array of traps formed by standing waves of light. The array contains millions of individual traps and isolates the molecules from one another, creating a pristine environment for measuring the eEDM.
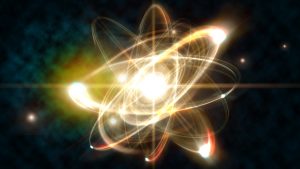
This measurement is made by watching how the spin of the molecules precesses in applied magnetic and electric fields, similar to the precession of a gyroscope or spinning top in a gravitational field. An electric dipole moment changes this precession rate, though only by the tiniest amount. That tiny change is detected more easily if the spin precesses for a long time. Because molecules are confined and isolated in the array, the time can be thousands of times longer than in current experiments. That makes my measurement scheme far more sensitive than any other experiment, allowing me to search for new physics with unprecedented precision.
How do you plan to consider things such as the motional magnetic field effect and the geometric phase in your experiments, given that these systematic effects have been important in previous electric dipole moment measurements?
An eEDM measurement at this extreme precision will require careful control of many potential systematic errors. In previous eEDM experiments using atoms, the motional magnetic field effect and the geometric phase had been major problems. Our team has previously showed the potential of the molecular method, particularly its insensitivity to motional magnetic fields. Potential systematic errors arising from geometric phases can be measured using different rotational states of YbF molecules, and they can then be controlled.
In addition to the two systematic effects, I expect to face other various systematic errors at this extreme sensitivity. These include the effects of magnetic fields and vector light shifts. To handle these systematic errors, one useful approach is to deliberately amplify various imperfections in the experiments, measure the effect, and then eliminate the uncertainty of the effect by controlling each imperfection to the required level. Using this approach, the systematic uncertainties scale with the statistical uncertainties, so will be helped by the vastly increased statistical sensitivity of the new apparatus. Again, I will further reduce various systematic effects by co-magnetometry using different rotational states of YbF molecule.
Given that you have only recently been awarded the Ernest Rutherford Fellowship, where will your first steps lie?
I have already taken the first step towards building the new apparatus. At Imperial College, I have completed the development of the required laser cooling technique and I am building a new cryogenic source of YbF molecules using a 1 Kelvin cryocooler. The first milestone, which I aim to achieve in the first year, is to decelerate the YbF molecules from the new source to rest using a laser slowing technique, before capturing them in a magneto optical trap.
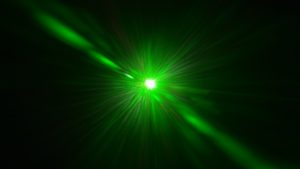
In addition to the scientific objectives, I have several other goals for myself, the academic community, and the public at large. The experimental method and precision measurement with an array of ultracold molecules will be a ground-breaking tool for related fields. Through presentations at seminars and conferences, I will ensure that the ideas and methods reach them.
Most importantly, with the Ernest Rutherford Fellowship I aim to strengthen ties between the AMO and particle physics communities by attending relevant national (e.g. PPAP) meetings and international workshops. These activities will also help to improve my visibility in the field of experimental particle physics and to build my leadership within the high energy physics community.
Outreach is an important component of my fellowship because searches for new particles is an area the public finds interesting and people are fascinated that this work can be done in small scale experiments. I have therefore arranged my first outreach activity: I will visit London Korean School and introduce primary school children to the ideas of atoms, light, and their interactions, and how they were produced in the early Universe.
What do you expect/hope to find from this work? What could perhaps lie beyond the Standard Model?
The success of my project will explore physics beyond the Standard Model up to 1,000 TeVac, so the research will be beneficial to the entire particle physics community. If I find a non-zero eEDM, which I hope for the most, then that will be direct evidence of physics beyond the Standard Model, providing valuable information on the requirements of new models. Alternatively, a null result at the proposed precision level will constrain the parameter spaces of many hypothesised theories. Therefore, I expect to provide important indicators of how the Universe was created during the early stages.
Dr Jongseok Lim
Research Fellow
Centre for Cold Matter
Imperial College, London
j.lim@imperial.ac.uk
Tweet @imperialcollege
www.imperial.ac.uk/centre-for-cold-matter/
Please note, this article will also appear in the seventh edition of our quarterly publication.