Dr Markus Rimann, head of Tissue Engineering for Drug Development (TEDD) at the University of Zurich, speaks to The Innovation Platform about the symbiotic nature of innovation in biomaterials and bioprinting.
The artificial production of tissues has been a dream for many scientists and clinicians for decades. In the early phases of tissue engineering, acellular scaffolds were implanted into the defective tissue site of patients to be repopulated by body cells to regenerate the defect. Thus, the name ‘tissue engineering’ mainly referred to applications in regenerative medicine. Although the expectations in tissue engineering were rather high, the progress in this field was quite slow. It was only with the advent of three-dimensional (3D) cell culture technologies that the field of tissue engineering received new impetus.
In the first decade of the 21st century, it became evident that cells grown in 3D better represent human physiology than cells grown in standard monolayer 2D cultures. With this further development of 3D cell cultures, the application range also broadened from regenerative medicine to drug development, substance testing and personalised medicine. In scaffold-based 3D cell cultures, biomaterials (scaffolds) are used to provide a 3D environment for the cells. Biomaterials developed for 3D cell culture are subdivided into synthetic or natural biomaterials based on their origin. Commonly used natural polymers include collagen I, Matrigel, fibrin, alginate, cellulose, hyaluronic acid, gelatine or mixtures thereof, whereas synthetic polymers are based on poly(ethylene glycol) (PEG), polyurethane and poly(vinyl alcohol).
For printing purposes, the rheological properties need to be considered and adapted accordingly. Currently, many of these biopolymers are modified to make them suitable for bioprinting. Bioprinting is mainly based on three technologies:
- Laser-assisted bioprinting;
- Inkjet-bioprinting; and
- (Micro-) extrusion-bioprinting.1
Bioprinting is expected to revolutionise tissue engineering because it allows tissue generation in an additive manner by the controlled deposition of cells, biomaterials and bioactive molecules in 3D space.
Bioprinting at ZHAW
In 2010, the ZHAW teamed up with the Swiss company regenHU. regenHU is a pioneer in providing bioprinting solutions for different applications in tissue engineering. The regenHU bioprinter installed at ZHAW laboratories was providing inkjet and micro-extrusion printheads. Inkjet printing allows cell deposition in small droplets in an aqueous solution. The advantage of inkjet printing is the high printing resolution of 20µm at the expense of printing speed. Furthermore, only aqueous cell suspensions can be printed to avoid print valve clogging.
On the other hand, micro-extrusion allows for the printing of viscous cell solutions, bioinks, as strands to generate different tissue layers. In collaboration with regenHU, ZHAW was producing a bioprinted full-thickness skin model supported by the Swiss funding agency Innosuisse (Grant Nos.: 12148.2 & 14331.1). The goal of the skin bioprinting project was to provide the cosmetic industry with advanced human skin models to address the hazardous potential of cosmetic ingredients, such as corrosion or irritation effects.
For the bioprinting process, a bioink was developed at ZHAW that was later commercialised by regenHU. The bioink consisted of gelatin and PEG. Gelatin, as a derivative of collagen I, provided cell adhesion sites and showed favourable printing properties at room temperature (RT) because of temperature-dependent polymerisation. PEG on the other hand, served as spacer between the gelatin molecules. In order to render the bioink stable at physiological conditions (37°C), the gelatin was methacrylated and was combined with methacrylated PEG. Polymer methacrylation allowed light-induced polymerisation with the help of a photoinitiator. The following protocol was developed to produce full-thickness skin models:
- The bioink without cells was printed in extrusion mode and each layer was photo-polymerised before
- Primary human dermal fibroblasts were printed with inkjet mode on the polymerised bioink layer
These steps were alternated eight times to generate a dermal equivalent (see Fig. 1). The dermal equivalent was incubated for up to several weeks to let the tissue mature and, afterwards, the epidermis was produced by placing primary human keratinocytes on top of the dermal equivalent. In this manner, we successfully printed a skin model.2 The approach was further developed and implemented at a cosmetic company. In another collaboration with the pharma industry, we developed a muscle-tendon tissue for drug development. Innosuisse supported the project (Grant Nos: 16313.1 & 27901.1). The bioink developed for the skin project served as material for the bioprinted muscle tissues.
As a prerequisite for the functional analysis of skeletal muscle tissues, a 24 well plate was developed to culture the tissues between two posts that were placed in each of the wells. Primary human skeletal muscle derived cells in combination with primary tenocytes (to represent tendon tissue) were printed to place tenocytes around the posts and muscle cells between the posts. This printing pattern was reflecting the in vivo-like tissue organisation, while tendon is attached to the bone (posts) and muscle tissue is between the tendon tissue and between the posts.
The main challenge of this project was the co-differentiation of the two cell types into the two corresponding tissues after the printing process. After the differentiation process into muscle fibres, the tissue was contracting after electrical stimulation proofing muscle functionality.3
Later, with an extended collaboration consortium we developed a device that electrically stimulated and at the same time optically monitored muscle tissue contraction through post bending under physiological conditions. In a proof-of-concept study, effects of know compounds could be reproduced (publication in preparation).
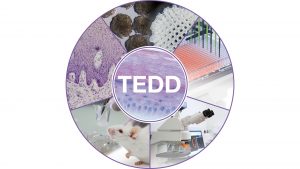
The TEDD network
While bioprinting technology is developing very quickly, the development of suitable bioinks is lagging behind. In the beginning, the idea was to develop a so-called ‘universal bioink’ to produce any kind of tissue. Today, it has become clear that there is no such universal biomaterial but that every tissue has its own cell microenvironment that needs to be reproduced to a certain extent. In order to develop specific bioinks, experts from the clinics, biology, chemistry, material sciences and engineering need to join forces. In 2010, the ZHAW together with the company InSphero founded the Competence Centre TEDD (Tissue Engineering for Drug Development and Substance Testing; TEDD) with the goal of further developing in vitro 3D cell culture technologies and its implementation in different industries (pharma, medtech, cosmetic) (see Fig. 2).
It is a collaboration platform to bring together different stakeholders from basic, applied and clinical research, enabling technology providers and industry to develop new solutions in the field. In 2017, the TEDD Annual Meeting was fully dedicated to latest advancements in bioprinting. During the meeting, new collaborations were initiated to boost the bioprinting field.
Conclusions and future directions
The ultimate goal of bioprinting is to produce artificial organs for organ transplantation, e.g. liver, kidney etc. So far, only small tissues have been bioprinted. One of the obstacles to generate larger tissues or even organs is the integration of blood supply. Thus, many research groups are working on the integration of the vasculature in the bioprinted tissues with increasing success. It will therefore just be a matter of time before we will have fully vascularised tissues/organs.
On other the hand, there are many applications in the field of drug development where blood supply is not important due to the small tissue size, with the benefit of being able to replace animal experiments, which is becoming increasingly demanded by society.
Finally, yet no less importantly, let us keep in mind that bioprinting is not the solution for every tissue-engineering problem. It is just a way to assemble cells in vivo-like in 3D, but without an additional incubation/maturation/differentiation step, a tissue will not be built.
References
- Jos Malda et al, ‘25th Anniversary Article: Engineering Hydrogels for Biofabrication’, Advanced Materials, 2013, DOI: 10.1002/adma.201302042
- Markus Rimann et al, ‘Standardized 3D Bioprinting of Soft Tissue Models with Human Primary Cells’, Journal of Laboratory Automation 1–14, 2015, DOI: 10.1177/2211068214567146
- Sandra Laternser et al, ‘A Novel Microplate 3D Bioprinting Platform for the Engineering of Muscle and Tendon Tissues’, SLAS Technology 1–15, 2018, DOI: 10.1177/2472630318776594
Dr Markus Rimann
Head of TEDD
Group Leader 3D Tissues and Biofabrication
ICBT Institute of Chemistry and Biotechnology
ZHAW Zurich University of Applied Sciences
+41 (0)58 934 55 12
markus.rimann@zhaw.ch
www.zhaw.ch/icbt/3d-tissues-and-biofabrication
Please note, this article will also appear in the second edition of our new quarterly publication.